Materials and Methods
Case Study:
Bioethanol from Enteromorpha intestinalis
The above-mentioned challenges are addressed in this case study,
which involves bioethanol production from green macroalgae; Enteromorpha
(Ulva) intestinalis belonging to Ulvaceae family.
They grow profusely and occupy intertidal zones under favourable
nutrient, salinity, light and temperature condition. E.
intestinalis is composed of 40.1% total carbohydrate,
20.4% Protein, 2.8% Lipid. Elemental analysis such as carbon
33%, nitrogen 4.36% and hydrogen 6.44% were recorded.
Biochemical composition of E. intestinalis is
comparable to earlier studies Cho et al., (2013) recorded 42.8%
carbohydrate, 31.6% crude protein and 1.3% crude lipid.
Bioethanol prospects from E. intestinalis is elucidated
in this section (Fig 6).
Fig. 6. Bioethanol production from green macroalgae
Enteromorpha intestinalis
Dilute acid hydrolysis of E. intestinalis at 0.7 N
H2SO4, 5% substrate concentration,
121oC for 45 min produced 239.94±1.3 mg/g of reducing
sugar. Enzyme extracted from marine bacteria Vibrio
parahaemolyticus (Hebbale et al., 2019). Pretreated
biomass of E. intestinalis subjected to enzyme
saccharification at pH 6, 50oC for 24 and obtained
reducing sugar of 289.89±2.4 mg/g of reducing sugar. Acid
pretreated macroalgal biomass was subjected to enzyme hydrolysis
using enzyme and incubated for 24 h, 1.2-fold increase in
reducing sugar was observed in E. intestinalis when
compared to dilute acid pretreatment. Scanning electron
micrographs of hydrolysed biomass indicates that the dilute acid
pretreatment prior to enzyme saccharification is a prerequisite
as it loosens the rugged surface of the biomass increasing the
surface area and exposing more of internal cellulose as seen in
Fig 7.
Fig 7. Scanning electron micrograph
of E.intestinalis illustrating
ultrastructural variations in the feedstock after
pretreatment and saccharification.
Acid hydrolysate obtained subjected to SHF using Pichia
kudriavzevii yeast strain isolated from Toddy juice at
35oC, 100 rpm for 24 h. Ethanol of 0.16 g with 51.8%
efficiency obtained. Pretreated biomass subjected to SSF process
using enzyme from Vibrio parahaemolyticus and Pichia
kudriavzevii yeast at 55oC,100 rpm for 24 h.
Ethanol of 0.10 g obtained with 65.1% efficiency. SSF exhibited
higher efficiency than SHF process.
Mass-energy balance was carried for analysing ethanol production
from P. kudriavzevii (TY) and the sugars obtained from
both SHF and SSF processes. Results obtained were extrapolated
to 1kg to make the study more comprehensive. Fermentation of
E. intestinalis in SHF process, obtained 23.9 g/kg
(30.4 ml/kg) ethanol with 55.9% conversion efficiency whereas in
SSF process 28.9 g/kg (35.8 ml/kg) ethanol with 83.9% conversion
efficiency was obtained (Table 6). Ethanol from SSF was
estimated 1.18-fold higher than the ethanol obtained from SHF
process indicating better efficiency. Similar results were
obtained for fermentation of E. intestinalis using S.
cerevisiae. SSF process achieved 30.5% efficiency when
compared to SHF (26.9%) process indicating better performance
regarding fermentation yield and faster process. Similar
mass-energy balance study was reported with the various
feedstock, 1kg of Saccharina japonica biomass yielded
23.1g (29.2 ml) of ethanol using SSF process achieving
conversion efficiency of 67.41% (Lee et al., 2013). Fermentation
of 1kg G, verrucosa produced 38g (48.1 ml) of ethanol
from SSF process achieving fermentation efficiency of 86% (Kumar
et al., 2013). Acid pretreatment of 1kg K. alvarezii followed
by detoxification produced 80g of galactose which was fermented
(SSF) to produce 43.7g (55.3 ml) of ethanol achieving 78.5%
conversion efficiency (Hargreaves et al., 2013). Fermentation of
1kg of switchgrass (second generation feedstock) produced 178.4
g (226.1 ml) of ethanol using SHF process, while SSF process
produced 183.5g (232.5 ml) of ethanol, but achieved lower
conversion efficiency which is attributed to the presence of
insoluble lignin in the biomass, that was treated using Ammonium
Fibre Expansion (AFEX) (Jin et al., 2010). Higher ethanol in SSF
process is due to the rapid consumption of glucose by yeast as
they were produced during enzyme hydrolysis (Xiao et al., 2004).
Acid hydrolysis of 1 kg of Lantana camara followed by
delignification, enzymatic hydrolysis of the biomass and
fermentation yielded 148.14 g (187.7 ml) of ethanol, whereas,
fermentation of pentose rich hydrolysate yielded 51.6 g (65.3
ml) of ethanol (Kuhad et al., 2010). Bagasse pith (1 kg)
(second-generation feedstock) produced 46.2 g (58.5 ml) and 66.4
g (84.1 ml) of ethanol by SHF and SSF process respectively. In
this study, commercial enzyme cellulase and β-glucosidase were
employed for enzyme hydrolysis and fermentation was carried
using P. stipitis JCM 10742 (Sritrakul et al., 2017).
Notable advantages were observed from SSF over SHF process, as
SSF process is amenable to enzyme hydrolysis with the rapid
ethanol production and occurrs in a single reactor, thereby
reducing the operation and investment costs for setting up a
biorefinery.
Ethanol production from macroalgal biomass results in large
quantity of spent biomass or waste products that are generally
disposed. High value products are created from these wastes
through the concept of biorefinery which aims to achieve no
waste flow resulting in economic and environmental benefits
(Balina et al., 2017).
Table 6. Ethanol
production from different macroalgal feedstock
(expressed in L per 100kg of biomass) |
Feedstock
|
Ethanol L per 100kg
|
1st generation feedstock
|
Corn grain |
35.6 |
Sorghum |
35 |
Cassava |
17 |
2nd generation feedstock
|
Rice |
43 |
Wheat |
34 |
Grapes |
13 |
Sugarcane bagasse |
76 |
|
Switchgrass |
22.6 |
3rd generation feedstock
|
Gracilaria verrucosa |
4.7 |
Ulva fasciata |
11.7 |
Kappaphycus alvarezii |
1.7-2.4 |
Gracilaria corticata |
2.6 |
Laminaria digitata |
26.2 |
Gelidium amnasii |
1.62 |
Enteromorpha intestinalis* |
3.55 |
Ulva lactuca* |
5.58 |
Palmaria palmata |
1.6 |
Ulva pertusa |
11.6 |
Lamanaria japonica |
36.8 |
Gelidium elegans |
23.2 |
Gelidium amansii |
8.8 |
Sargassum sp. |
28.70 |
*Present study |
|
|
Economic
Prospects of Macroalgae- Biorefinery
Seaweeds were mostly restricted to domestic purposes such as food
and feed, preparation of industrial gels, medicinal uses such as
Laminaria sp. for dilation of cervix in difficult
childbirth, Gelidium sp. used for intestinal
afflictions etc. In recent times, macroalgal biomass are
cultivated on largescale for production of more valuable
commodity than food and feeds. These include, extraction of
polysaccharides for agronomic applications, cosmeceuticals,
nutraceuticals, pharmaceuticals, and bioenergy. Seaweed
biorefinery approach extracts most valuable components from the
macroalgal biomass without altering the residue for commodity
purposes such as food, feed, fertilizers etc.(Balina et al.,
2017; Buschmann et al., 2017).
Macroalgae is subjected to dilute-acid pretreatment and
pretreated biomass is hydrolysed using enzyme. Enzyme
hydrolysate is fermented to produce ethanol. Solid/liquid
separation is carried out for the fermentation broth. The liquid
fractions are rich in lipid, minerals and other unutilized
sugars and are used as liquid fertilizers, which substitutes the
conventional mineral fertilizers. The solid fraction is spray
dried and rich with protein and minerals and is being used as
fish feed, which serves as a substitute for soy protein (Fig 7).
Selection of appropriate macroalgal feedstock accumulating
higher carbohydrate fractions and nutrients can lower the
CO2 level and provide climate change and marine
eutrophication mitigation services (Seghetta et al., 2016).
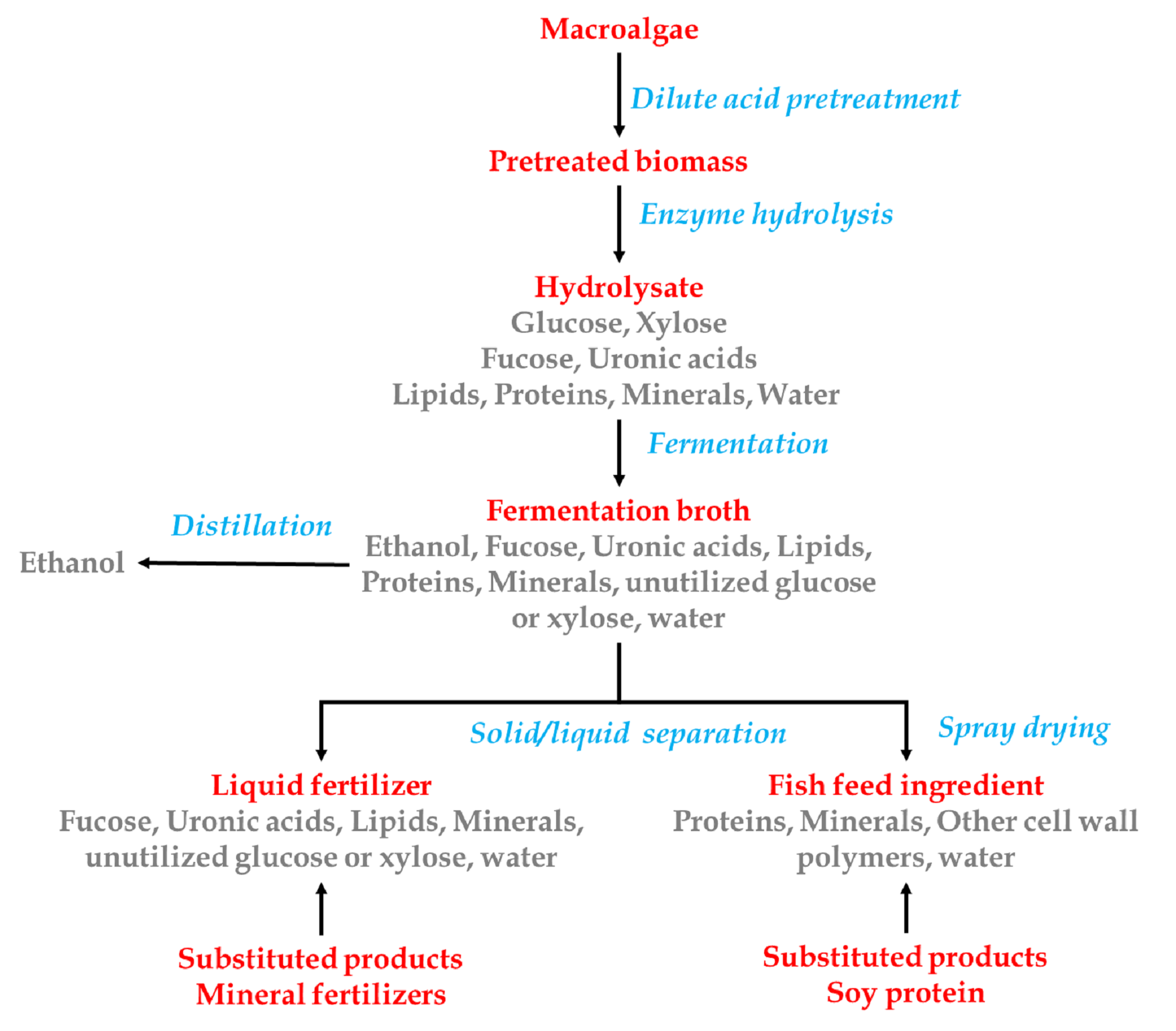
Fig
7. Seaweed biorefinery with probable constituents
Fatty acids content in the dried and
canned macroalgae are of linear structures and are major sources
of essential fatty acids such as palmitic acid and ω-3, 6 and 9.
Agar from G. edulis, G. acerosa and
Gracilaria sp. are extracted by boiling the seaweed and
extract is filtered, freeze thawed and dried in sun and market
as powder (Kaladharan and Kaliaperumal, 1999). Macrocystis
pyrifera was harvested for production of acetone and
potash (Roesijadi et al., 2010). Macroalgal biomass is composed
of high amount of water-soluble potash, which is readily
absorbed by the plants. Composting of seaweed along with shark
liver sediments and fish offal (15:4:3 by weight) fetches high
manure value with 2.4% N, 0.7% P and 3.5 % potash (Chennubhotla
et al., 1981). Macroalgal biomass are regarded as ‘super foods’
for being rich in vitamins B12, A and iodine. Seaweed meal
incorporated in poultry and animal feed increased the iodine
content of the eggs and milk production in dairy cows (Hebbale
et al., 2017; Holdt and Kraan, 2011; Torres et al., 2019).
Discarded waste of algin extracted macroalgal biomass is
estimated to contain 93-94% of iodine (Torres et al., 2019).
Extracted protein fraction from Ulva increase illeal
digestibility and rumen fermentation (Baeyens et al., 2015;
Bikker et al., 2016). Apart from whole seaweed, residue obtained
from industries; floating residues, spent biomass serves as
feedstock for bioethanol production (Sudhakar et al., 2016).
Therefore biorefinery approach is sustainable and
environmentally friendly as it reduces the burden on
environment.
Fatty acids content in the dried and canned macroalgae are of
linear structures and are major sources of essential fatty acids
such as palmitic acid and ω-3, 6 and 9. Agar from G. edulis,
G. acerosa and Gracilaria sp. are extracted by
boiling the seaweed and extract is filtered, freeze thawed and
dried in sun and market as powder (Kaladharan and Kaliaperumal,
1999). Macrocystis pyrifera was harvested for
production of acetone and potash (Roesijadi et al., 2010).
Macroalgal biomass is composed of high amount of water-soluble
potash, which is readily absorbed by the plants. Composting of
seaweed along with shark liver sediments and fish offal (15:4:3
by weight) fetches high manure value with 2.4% N, 0.7% P and 3.5
% potash (Chennubhotla et al., 1981). Macroalgal biomass are
regarded as ‘super foods’ for being rich in vitamins B12, A and
iodine. Seaweed meal incorporated in poultry and animal feed
increased the iodine content of the eggs and milk production in
dairy cows (Hebbale et al., 2017; Holdt and Kraan, 2011; Torres
et al., 2019). Discarded waste of algin extracted macroalgal
biomass is estimated to contain 93-94% of iodine (Torres et al.,
2019). Extracted protein fraction from Ulva increase
illeal digestibility and rumen fermentation (Baeyens et al.,
2015; Bikker et al., 2016). Apart from whole seaweed, residue
obtained from industries; floating residues, spent biomass
serves as feedstock for bioethanol production (Sudhakar et al.,
2016). Therefore biorefinery approach is sustainable and
environmentally friendly as it reduces the burden on
environment.
Scope for further research
Marine macroalgae have been explored worldwide for various
application owing to their ability to accumulate large
concentration of biomolecules (especially carbohydrates), which
serve as raw material for bioethanol production and other
value-added products. Bioprocess of bioethanol production
involves three major steps; dilute acid pretreatment, enzyme
saccharification and fermentation. The major future prospective
for bioethanol production from macroalgal biomass includes (i)
exploring enzymes having higher catalytic activity and stability
at extreme conditions (ii) yeast microorganisms able to ferment
broad range of sugars (iii) improved ethanol yield by process
optimization (iv) Consolidated bioprocess involving cellulolytic
yeast to hydrolyse cellulose as well as fermented subsequent
glucose released during hydrolysis to ethanol. Biorefinery
approach can be realised only with sufficient quantities of
biomass. Largescale cultivation of macroalgae in open ocean is
results is disease outbreaks and destruction of habitat (killing
endemic corals) (Bindu & Levine, 2011; Patterson et al.,
2008). In order to overcome this, Integrated Multi-Trophic
Aquaculture (IMTA) (Fig. 8) concept is introduced, which farming
macroalgae in close proximity to other species at different
trophic level on land. Land-based seaweed cultivation with
adaptation to much wider range of macroalgal genera offers raw
materials for higher-value product development. Intertidal
species like Ulva sp. and Enteromorpha sp.
have high tolerance to temperature and irradiance ranges, which
can be cultivated in IMTA system. Cultivation of seaweeds for
biofuel productions needs to be encouraged to meet the future
fuel demand as seaweeds have high potential as feedstock for
biofuel production as part of the Nation’s strategic energy
security program. This would also empower rural women with job
opportunities. Development of seaweed-based industries at
decentralized levels along coastal areas, where resources are
abundantly available would enhance the job opportunities for the
rural youth. Seaweed cultivation as a notable future enterprise
can open up platform for establishing seed hatcheries, seeding
units and processing units and enhance employment opportunities
in rural coastal area
Fig. 8. Integrated
Multi-Trophic Aquaculture Model
|