Introduction
Major commercial energy sources such as oil, coal and natural gas are extracted from fossil fuels. Burning of fossil
fuel results in the escalation of CO2 in the atmosphere- a major cause for global warming, price
volatility, air pollution and environmental degradation (Adenle et al., 2013; Naik et al., 2010). Surging demand in
these sectors has led to increase in oil production from the finite source of fuel reserves. Continuous exploitation
is depleting these reserves at staggering speed and will no longer suffice the world’s energy demand (del Río et
al., 2020; Goli et al., 2016; Hirsch et al., 2005; Raheem et al., 2018) leading to global energy crisis. Hence,
fossil fuels are regarded as unsustainable and questionable from economic, ecology and environmental point of view
(Naik et al., 2010). Therefore the quest for an economical, renewable, sustainable and environmentally benign source
of energy is underway (Hahn-Hägerdal et al., 2006; Tripathi et al., 2016). Biomass energy in the form of cow dung
cake, firewood, agriculture residue and other natural feedstock for cooking and heating have been prevailing from
ages which contributes to 80% of rural energy in developing countries like India (Kumar et al., 2015; Ramachandra,
2010 , 2000, 2004). Biofuels from biomass such as plants, algae or organic waste are emerging as promising
alternative renewable energy sources to liquid fuels ((Jambo et al., 2016). Different technologies have evolved
towards the conversion of biomass into fuels and other value added products that have the advantage of mitigating
global warming by cutting down the carbon dioxide emission, as CO2 is fixed by the biomass via
photosynthesis, making it a carbon neutral emission (del Río et al., 2020) and also ease the dependency on oil
reserve (Bhattacharyya, 2006; Kumar et al., 2015; McKendry, 2002; Naik et al., 2010). Biofuels are of two types
bioethanol and biodiesel; bioethanol are produced from carbohydrate rich algal biomass (e.g. macroalgae) whereas,
biodiesel is produced from lipid rich algal biomass (e.g. microalgae). Dependence on fossil fuels (gasoline) in
transport sector can be reduced by bioethanol, as it is effective in replacing or blending with gasoline.
Development and commercialization of bioethanol is largely achievable due to the availability of feedstock in large
quantum (Jambo et al., 2016). Bioethanol feedstocks are categorised into first, second and third generation based on
the feedstock’s carbon source. Bioethanol from first generation feedstock (1G) involved food crops like corn and
sugarcane, which encountered resistance due to the arable land, freshwater source for its cultivation and
competition with food crops (Naik et al., 2010). The lacunae of 1G bioethanol in supplementing the growing energy
demand led to the exploration of alternate feedstock involving agricultural residues and woody biomass rich in
lignocellulose (second generation (2G) bioethanol feedstock). However, 1G and 2G bioethanol production failed, due
to process technology involving the cost-intensive delignification process and difficulty in scaling up (Zhu and
Pan, 2010). Bioethanol potential from 1G and 2G feedstock marginally complies with various other sustainability
criteria’s such as; conversion of ecologically vulnerable wetlands, extensive usage of fertilizers, soil erosion,
rainforests, peat lands, savannas into energy crop lands, disruption of global food supply contributing to several
magnitude of CO2 (Gasparatos et al., 2013; Maeda et al., 2015). Bioethanol production from third
generation feedstock (3G) involves algal biomass that are grown in fresh water, wastewater (Ramachandra et al.,
2013) and marine waters with zero nutrient input and more importantly, non-interference with the lands required for
food production (Demirbas, 2008; Odum and Heald, 1972). At present, the research focus is currently on bioethanol
production from 3G feedstock due to higher photosynthetic efficiency (6-8%), productivity (~13.1 kg dry
weight/m2 over 7 months), ease of cultivation, low consumption of fertilizers, no alteration with food
supply, and high absorption of CO2 (8-10 tonne CO2 per hectare) (Kraan, 2013),
potential to obtain high value added products (pigments, cosmetics, food additives etc.). Algal biomass has emerged
as one of the ideal feedstock for achieving sustainable biorefinery having immense potential for commercialization
(Jambo et al., 2016).
|
Fig.1. Bioethanol production process from biomass |
Bioethanol production from algal biomass
Production of bioethanol from algal biomass involves three steps; pretreatment, saccharification and fermentation
which are discussed in detail in the subsequent sections. Algae are of two types - micro and macroalgae. Microalgae
are explored for production of biodiesel (Ramachandra et al., 2009; Saranya et al., 2018), whereas macroalgae rich
in carbohydrate are suitable for production of bioethanol (Borines et al., 2013; John et al., 2011; Ramachandra
& Hebbale, 2016; Ramachandra & Hebbale, 2020; Roesijadi et al., 2010; Wei et al., 2013; Yanagisawa et al.,
2013). Macroalgae (commonly known as seaweeds) are multicellular, photosynthetic algae growing in marine environment
and lesser extent in brackish waters. Photosynthetic pigments in seaweeds imparts characteristic range of colors
such as red (Rhodophyta), green (Chlorophyta) and brown (Phaeophyta) algae (Abbott et al., 1992; Smith, 1938; Van
Den Hoek, 1984). Green seaweeds are euryhaline i.e. tolerating a wide range of salinity whereas, red and brown
seaweeds are strictly marine dwelling. Seaweeds have a wide range of distribution from tropics, temperate, polar,
tidal pools, estuaries, deep waters and rocky shores whereas, brown seaweed species belonging to order Laminariales
occur mostly in temperate regions (<24oC) (Abbott et al., 1992). Seaweeds
grow by attaching to a substrate (natural or artificial); for need of stable anchorage large seaweed beds are
restricted to rocky substrate (Abbott et al., 1992; Speight and Henderson, 2013). Macroalgal tissues lack
specialized translocatory systems and structure of the “higher plants” (Abbott et al., 1992). Macroalgal body is
rootless, stemless and leafless entity called thallus, although many have superficially leaf-like blades, stem-like
stipes and often having attaching organs called holdfast or haptera (Lobban et al., 1994; Smith, 1938). Most algae
lack these structures owing to their morphological adaptations and modifications (Abbott et al., 1992). Seaweeds
reproduce either asexually or sexually (Lobban et al., 1994). Asexual reproduction is a common mode of reproduction
in seaweeds.
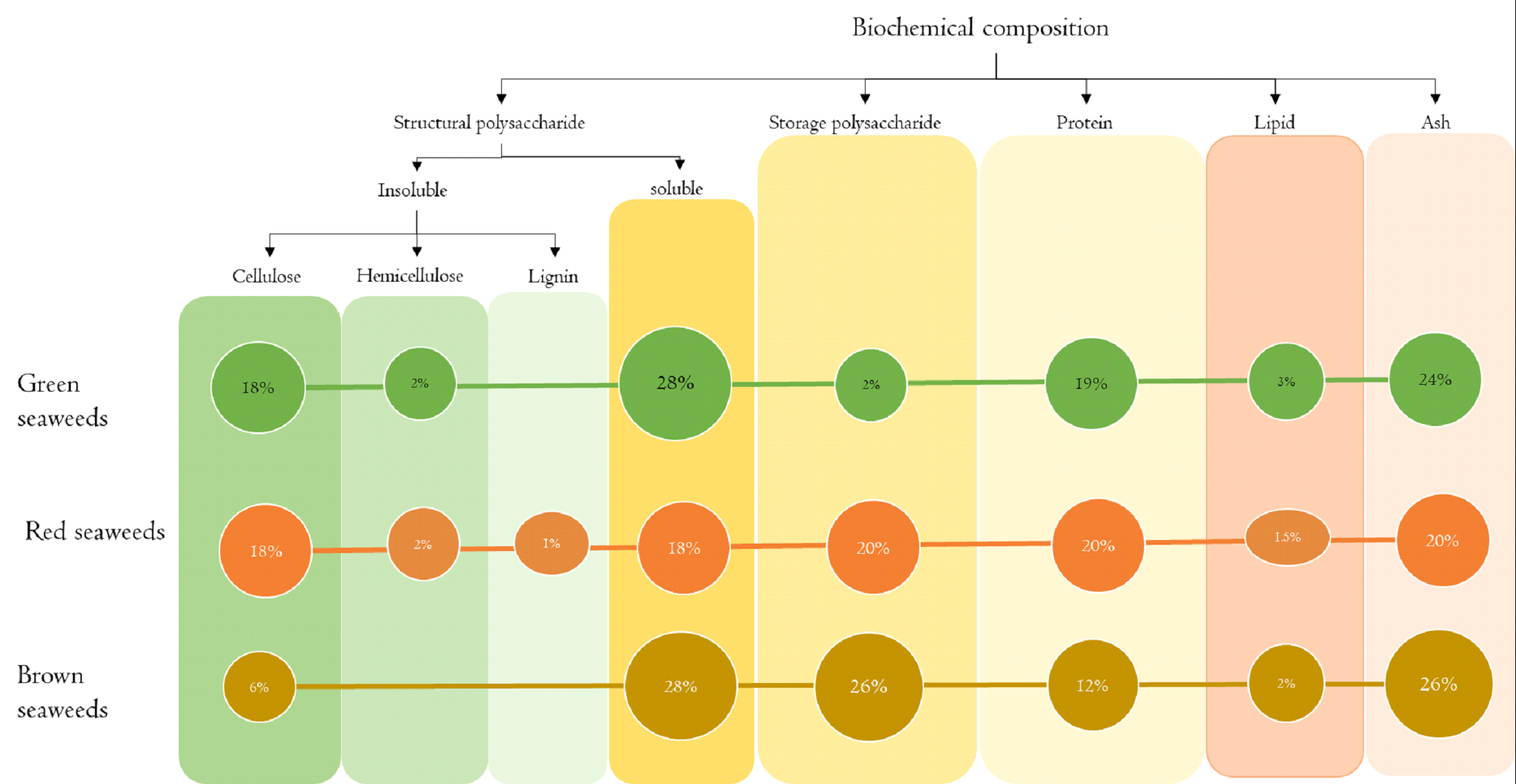
Fig.2. Macroalgal biochemical composition profile
Generally, the range of biochemical composition of seaweeds are; carbohydrate: 25-77% dry weight, proteins: 5-43% dry
weight, lipid: 1-5% and ash content: 9-50% dry weight followed by higher water content of 70-90% fresh weight (Jung
et al., 2013; Praveen et al., 2019). Seaweeds consist of varied profiles of structural and storage carbohydrate
(Daroch et al., 2013; Kostas et al., 2016) based on the respective intercellular spaces and cell wall (Pereira and
Neto, 2014) (Fig 2).
Seaweed polysaccharides show a range of structures and fulfil a variety of functions similar to neutral sugars and
sugar acids of terrestrial plants. Certain seaweeds also contain acidic half ester sulphated groups attached to
hydroxyl groups of sugars. Hexose sugars such as glucose, galactose and mannose found in these polysaccharides have
identical chemical composition. Carbohydrate reserves of red algae are usually stored in the form of small grains
that lie in the cytoplasm outside the algal plastids, the chromatophores. The insoluble carbohydrate reserve of red
algae has been called floridean starch (intermediate between true starch and dextrin) (Yu et al., 2002).
Polysaccharides such as starch and cellulose in green algae are similar to that of terrestrial plants. Macroalgal
biomass lacks lignin in their composition (Jung et al., 2013) except in few red seaweed species. Apart from higher
content of carbohydrates in seaweeds, protein and ash contents are also relatively higher; however, lipid fraction
is considerably low.
An effective bio-refinery process is achieved by characterization of the feedstock employed such as large variety of
carbohydrate (mono-, di-, polysaccharides) serves as raw materials for bioethanol production. Quantification of
carbohydrate content (Table 1) in the biomass is an essential step in biorefinery process as it is directly
proportional to ethanol yields in biochemical conversion process and facilitates overall process efficiency
calculations as well as mass balance (Aden et al., 2002; Kostas et al., 2016). Seaweeds accumulate large
concentrations of carbohydrates (polysaccharides) made up of various monosaccharides such as xylose, glucose,
galactose and fructose. These sugars are converted to bioethanol through fermentation via appropriate
microorganisms.
Availability of macroalgal feedstock
Macroalgae occur along the nutrient rich coastal zones by attaching on hard substratum. Global seaweed distribution
is highest between 60oN and 60oS latitude with 900-1100 species, least species are recorded
>60o in both hemispheres, in these regions, mostly cold water desiring macroalgae are recorded such as
Laminaria, Undaria etc. (Hurd et al., 2014). The most prominent macroalgal genera explored for
bioethanol potential is indicated in Fig 3. along the coastal regions of India.
Table 1. Biochemical composition and monosaccharide profile of potential
macroalgal genera for bioethanol production |
Biochemical composition (%)/ 100 g dry biomass |
Ulva sp. |
Gracilaria sp. |
Gelidium sp. |
Sargassum sp. |
Kappaphycus sp. |
Laminaria sp. |
Ash |
18-49.6 |
22.9-26 |
2.5-4.8 |
40.0-46.0 |
18.0-19.7 |
8.7-41.2 |
Lipid |
1-3.5 |
0.7-6 |
0.7-7.4 |
0.75-2.5 |
0.2-0.75 |
0.6-3.4 |
Protein |
10.7-25.9 |
4.3-16 |
10.2-18.7 |
10.25-15.42 |
2.3-5.74 |
1.1-19.8 |
Carbohydrate |
53-69.9 |
30.4-76.67 |
53.2-75.8 |
23.5-41.81 |
51.6-59.58 |
33.9-76 |
Monosaccharides composition/ 100g carbohydrate |
|
3,6-Anhydrogalactose |
|
|
28.9-43.5 |
|
|
|
Arabinose |
0.0-0.8 |
|
|
|
|
|
Fucose |
0.2-0.4 |
|
33.6-57.0 |
0.2-7.4 |
|
4.2-8.5 |
Galactose |
7.2-8.5 |
30.6-42.8 |
21.8-40.6 |
0.0-4.0 |
20.3-22.39 |
|
Glucose |
0.2-25.4 |
20.5-24 |
|
22.5-65.6 |
0.4-0.78 |
24.5-62.2 |
Mannitol |
|
|
0.0-0.8 |
0.2-5.04 |
|
11.2-37.9 |
Mannose |
0.0-4.2 |
0.0-0.07 |
0.0-0.4 |
0.0-4.0 |
|
|
Rhamnose |
3.3-12.7 |
|
|
|
|
|
Ribose |
0.1-2.7 |
|
|
|
|
|
Uronic acids |
25.9-28.8 |
|
|
|
|
20.8-41.6 |
Xylose |
5.6-14.4 |
0.0-0.3 |
0.0-1.3 |
0.5-19.4 |
|
6.0-12.0 |
Bioethanol (L/100kg dw) |
5.58-11.7 |
2.6-4.7 |
1.62 |
4.4 |
1.7-2.4 |
5.4-26.2 |
(Abd-Rahim et al., 2014; Borines et al., 2013; Chennubhotla et al., 1990; Masarin et al.,
2016; Parthiban et al., 2013; Sung-Soo Jang, 2012; Wu et al., 2014; Yeon et al., 2011) |
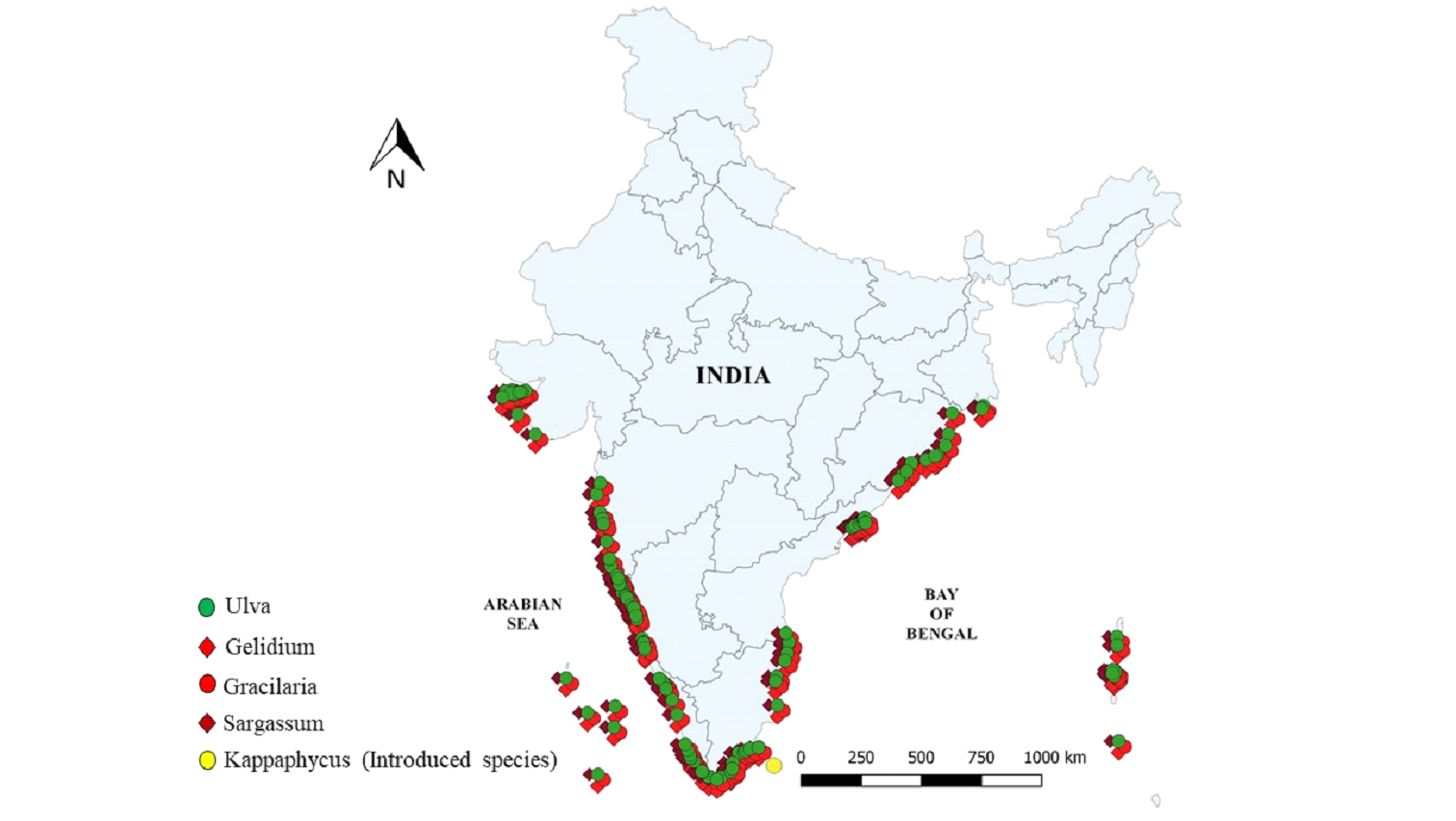
Fig. 3. Distribution of macroalgal species along the coastal regions across the India,
explored for bioethanol production potential ( Ramachandra & Hebbale, 2020)
Pretreatment
Bioethanol production from macroalgae requires extraction of fermentable sugars, several studies have reported (Table
2) of different pre-treatment techniques including chemical, physical or biological or combination of these
techniques through which higher sugar concentration can be obtained (Feng et al., 2011; Kim et al., 2014; Meinita,
Kang, et al., 2012; Park et al., 2012; Yoon et al., 2010). Pretreatment of biomass is carried out to reduce the size
and alter or remove structural and compositional impediments prior to subsequent enzyme hydrolysis. Pre-treatment
needs to be cost effective and release higher quantum of sugar with the minimal inhibitor formation.
Table 2. Assessment of selected pre-treatment processes |
|
Pre-treatment process |
Yield of fermentable sugars |
Physical or physico-chemical
Pre-treatments |
Mechanical |
Low |
Steam explosion |
High |
Ammonia fibre explosion (AFEX) |
Moderate |
Carbonic acid |
Very high |
Chemical pre-treatments |
Dilute acid |
Very high |
Concentrated acid |
Very high |
Alkaline extraction |
Very high |
Wet oxidation |
High |
Organosolvent |
Very high |
Biological pretreatments |
Commercial Enzymes or bacterial/fungal enzymes |
Very high |
Source: (Hendriks and Zeeman, 2009; Wooley et al., 1999) |
Most commonly used chemical pretreatment method for obtaining higher fermentable sugars from macroalgal biomass is
dilute acid pretreatment method, that employs mineral acids such as H2SO4 and HCl at milder
concentrations of 0.3-0.9N (Meinita et al., 2012a; Park et al., 2012). During dilute acid pretreatment process,
reaction parameters such as reaction time, acid concentration, and substrate concentration is involved for efficient
sugar release from algal feedstock (Table 3). Pre-treatment with dilute- H2SO4 at optimal
concentration and temperature is reported effective for cell-wall depolymerization. The advantage of dilute acid
pretreatment method is lower energy consumption as compared to other pre-treatments. However, disadvantage of dilute
acid pretreatment is formation of fermentation inhibitors such as 5-Hydroxymethyl furfural (HMF) and levulinic acid
(LA) with the degradation of hexose sugars and furfurals from pentose sugar degradation. Hence, enzyme hydrolysis
has been opted as sustainable option for hydrolysis as it does not involve formation of any
inhibitors because enzymes do not cause degradation of monosaccharides (Yanagisawa et al., 2013).
Table 3. Reducing sugar yield reported from macroalgal feedstock at different dilute
H2SO4 concentration |
Macroalgal species |
Dilute H2SO4 concentration |
Reducing sugar yield |
Reference |
Gracillaria verrucosa |
1.5% |
430 mg/g |
(Kumar et al., 2013) |
373 mM |
7 g/ l |
(Nguyen et al., 2017) |
0.1 N |
7.47 g/L |
(Kim et al., 2015) |
Kappaphycus alvarezii
|
0.9 N |
300 mg/g |
(Khambhaty et al., 2012) |
1% v/v |
81.62 g/L |
(Hargreaves et al., 2013) |
0.2 M |
30.5 g/L |
(Meinita et al., 2012) |
Laminaria japonica |
0.06% |
29.09% |
(Lee et al., 2013) |
Gelidium amansii |
3% |
33.7% |
(Park et al., 2012) |
Enzyme saccharification
Biological pretreatment method employs substrate specific enzymes (Fig 4). Major portion of macroalgal cell wall is
composed of cellulose, which is made up of glucose subunits, in order to break the cellulose structure cellulase
enzyme is commonly used. Similarly, agarases is used for agar, carageenase for carrageenan, alginase for alginate
and laminarases for laminarin. Pretreatment is a prerequisite prior to enzyme saccharification, as it opens up the
cellulose fibrils and maximizes the enzymatic conversion of cellulose (Harun, 2011; Jeong et al., 2013; Kang et al.,
2013; Kim et al., 2014). Commercial enzymes as well as enzymes extracted from bacteria or fungi are reported for
enzyme saccharification of macroalgal biomass (Table 4).
Enzyme saccharification of cellulose to glucose is considered an environmentally friendly pretreatment process.
However, this research is at a nascent stage, orientated toward isolating efficient enzyme systems (Swain et al., 2017)
from microorganisms that produce cellulolytic enzymes in their metabolic processes (Bhat and Bhat, 1997; Niehaus
et al., 1999; Zhang and Kim, 2010). Higher concentrations of extracellular cellulase enzymes have been reported from
bacteria and fungi that are feasible for large-scale production. Terrestrial sources for cellulase enzyme have been extensively explored and investigated; however, studies related to cellulase extraction from marine source is still an unexplored platform. A large reservoir of microbes thrives in the marine ecosystem at extreme conditions of salt,
temperature, and high pressure (Trivedi et al., 2016), which imparts well-developed cellular machinery and
stable enzymes, offering novel biocatalysts with unusual properties which can be explored for bioethanol production
(Gao et al., 2010; Zhang and Kim, 2010).
15.2.4 Fermentation
Sugars obtained from dilute acid hydrolysis, enzyme saccharification, or a combination of both are subjected to fermentation, where microorganisms consume the sugar as their sole source of carbon and metabolize it for their growth and
reproduction and yield ethanol as a by-product. Fermentation is dependent on the simple sugars; seaweeds consist of
both C6 and C5 sugars, but not all the microorganisms can metabolize both the sugars simultaneously. Hence the choice
of the organism for fermentation plays a pivotal role. The most widely used microorganism for ethanol fermentation is
Saccharomyces cerevisiae, which metabolizes hexose (C6) sugars. Fermentation of glucose alone will not produce high
yields of ethanol. Pichia stipitis and Pichia angophorae can metabolize pentose (C5) sugars. Other than yeast microorganisms, bacteria such as Pacchysolan tannophilus and Escherichia coli have also been studied for ethanol production
from hexose and pentose sugars. Macroalgae are also composed of sugar alcohols that are not metabolized by yeast
microorganisms; Zymobacter palmae isolated from palm was observed to convert mannitol present in brown algae into
ethanol (Horn et al., 2000a,b).
Glucose is metabolized in a series of enzyme-catalyzed reaction processes called glycolysis to yield two molecules
of three-carbon compound pyruvate. Under hypoxic or anaerobic conditions, pyruvate is decarboxylated, and acetaldehyde is reduced to ethanol through alcohol dehydrogenase (Nelson and Michael, 2008). Xylose is converted to xylulose
and phosphorylated to xylulose-5-phosphate and further metabolized to glyceraldehyde-3-phosphate and fructose-6-
phosphate, which then enters the glycolysis pathway for subsequent pyruvate and ethanol production (McMillan, 1993),
as illustrated in Fig. 15.5.
S. cerevisiae is the predominant microorganism utilized in ethanol fermentation in industrial bioethanol production
processes. Ethanol is produced via homoethanol pathways, by EmbdenMeyerhofParnas (EMP) glycolytic pathway,
|
Fig 4. Schematic representation of enzyme action on substrate |
Table 4. Reducing sugar yield reported from macroalgal feedstock using enzymes |
Macroalgal Feedstock |
Enzymes hydrolysis |
Sugar yield |
Reference |
E. intestinalis |
Viscozyme L and Cellic CTec2 |
20.1 g/L |
(Kim et al., 2014) |
|
Celluclast 1.5 L and Viscozyme L |
40 g/L |
(Cho et al., 2013) |
|
|
U. fasciata |
Cellulase 22119 |
215 mg/g |
(Trivedi et al., 2013) |
|
Viscozyme L |
206 mg/g |
|
Cellulase isolated from Cladosporium sphaerospermum |
112 mg/g |
( Trivedi et al., 2015) |
|
U. pertusa |
Meicelase-Simple saccharification |
43 g/L |
(Yanagisawa et al., 2011) |
|
|
Meicelase |
78.8 g/L |
|
Meicelase |
59.1 g/L |
|
Cellulase & Amyloglucosidase |
26 |
(Choi et al., 2012) |
|
|
G. elegans |
Meicelase |
70.9 g/L glucose |
(Yanagisawa et al., 2011) |
|
53.2 g/L galactose |
|
G. amnasii |
Cellulase 0.98 FPU/g β-glucosidase 10.4 U/g |
43.7% glucose |
( Kim et al., 2015) |
|
12% galactose |
|
K. alvarezii |
Celluloclast 1.5 L & Novozyme |
11 g/L |
(Tan and Lee, 2014) |
|
Multifect |
81 g/L |
(Hargreaves et al., 2013) |
|
G. amansii |
Enzyme Viscozyme L |
2.4 g/L |
(Ra et al., 2013) |
|
G. amansii |
Celluclast (0.168 EGU/ml) |
10.5 g/L |
|
G. verrucosa |
10% enzyme extract |
7.47 g/L |
(Kim et al., 2015) |
|
A. crassifolia |
Meicelase |
66.3 g/L |
(Yanagisawa et al., 2011) |
|
|
S. japonica |
Enzyme Cellulase- 45 FPU/g Cellobiase-55 CBU/g |
268.5 mg/g |
(Ge et al., 2011) |
|
U. pinnatifida |
Celluclast 1.5 (4ml/100g of cellulose) Novozyme 188 |
65 mg/g |
( Lee et al., 2011) |
|
Sargassum. sp. |
10 FPU cellulase /g ,250CBU cellobiase/g |
120 mg/g reducing sugar |
(Borines et al., 2013) |
|
S. japonica |
Novozyme (Termamyl 120L) |
20.6±1.9 g/L |
(Jang et al., 2012) |
|
Saccharomyces cerevisiae, is the predominant microorganism utilized in ethanol fermentation in industrial
bioethanol production processes. Ethanol is produced via homoethanol pathways, by Embden-Meyerhof-Parnas (EMP). The
EMP (glycolytic) pathway, summarized below (Walker & Walker, 2011):
Glucose + 2ADP + 2Pi + 2NAD+->Pyruvate + 2ATP + 2NADPH + 2H+
Saccharomyces cerevisiae reoxidizes the reduced co-enzyme NADH to NAD+ in terminal fermentative
step reactions emanating from pyruvate:
2Pyruvate + 2NADH + 2H+->NAD+ + 2Ethanol + 2CO2
The intermediate compound, acetaldehyde, acts as the electron acceptor
NAD+ is re-generated by alcohol dehydrogenase which requires zinc as an essential co-factor for its activity.
Fermentation thus maintains the redox balance by regenerating NAD and keeps glycolysis proceeding. In doing so,
yeast gets energy for its own maintenance by generating 2ATP. The theoretical (stoichiometric) conversion to ethanol
from glucose is as follows:
Fig.5. Glucose and Xylose metabolism and conversion to
ethanol
For each kilogram of glucose fermented, around 470 g of ethanol can be produced (i.e., <50%) representing a yield
of 92% of theoretical maximum. In industrial fermentation practice, however, the best yields are only around 90% of
this theoretical conversion due to the diversion of fermentable carbon to new yeast biomass and minor fermentation
metabolites (organic acids, esters, aldehydes, fuel oils etc). Bioethanol production from macroalgal biomass is
carried out either by Separate Hydrolysis and Fermentation (SHF) or Simultaneous Saccharification and Fermentation
(SSF) process. In SHF process, dilute acid hydrolysis/enzyme saccharification and fermentation are carried out
separately. This process involves higher operating cost, energy consumption and more reaction time. Not all the
sugars in the medium is utilized at the end of this process. In SSF process, enzymatic saccharification and
fermentation is achieved in the same reactor. This process is favourable as it requires slower process time, lower
energy consumption and yield higher ethanol. However, the process time required for both enzyme and yeast
microorganisms are different which results in slower release and consumption of sugar. Lower concentrations of
inhibitors are formed in SSF process.
Current Status
Kappaphycus, Gelidium, Gracilaria, Sargassum, Laminaria and Ulva, are largely cultivated
macroalgal genera for hydrocolloid extraction and human food usage by China, Philippines and Indonesia. However in
recent years these genera are regarded as potential feedstock for biofuel production in addition to the value added
products for phycocolloids extraction, human food, cosmetics, fertilizer and other chemicals (Harun, 2011; Jang,
Cho, Jeong, & Kim, 2012, Dhargalkar & Pereira, 2005; McHugh, 2003; Yanagisawa et al., 2013). Species from
these genera have been chosen considering the availability and assessment of resources around the globe, ease of
cultivation and harvesting. Shorter life cycles of seaweed are taken into advantage for largescale cultivation,
which is cost-effective and involves environmentally friendly methods, zero input of fertilizers, and no changes in
land use as they are exclusively grown in marine waters. Laminaria is most cultivated seaweed with average
production of 5.14 million tonnes (Alaswad et al., 2015).
Table 5. Current status of seaweed utilization |
Species |
Food |
Feed |
Industrial |
Medicine |
Fertilizer |
Biofuel feedstock |
Ulva fasciata* |
+ |
+ |
- |
+ |
- |
+ |
Enteromorpha compressa* |
+ |
+ |
- |
+ |
- |
- |
Enteromorpha intestinalis* |
+ |
+ |
- |
+ |
+ |
+ |
Monostroma oxyspermum |
+ |
+ |
- |
- |
- |
- |
Cladophora fascicularis* |
+ |
+ |
- |
- |
- |
- |
Chaetomorpha media* |
+ |
+ |
- |
- |
+ |
- |
Codium fragile |
+ |
+ |
- |
+ |
- |
- |
Caulerpa sertularioides |
+ |
+ |
- |
- |
- |
- |
Dictyota dichotoma* |
+ |
+ |
+ |
- |
- |
+ |
Spatoglossum asperum* |
- |
- |
+ |
- |
+ |
+ |
Hydroclathrus clathratus |
- |
- |
+ |
- |
+ |
- |
Stoechospermum marginatum |
- |
- |
+ |
- |
+ |
- |
Colpomenia sinuosa |
- |
- |
+ |
- |
+ |
- |
Dictyopteris australis |
- |
- |
+ |
- |
+ |
- |
Padina tetrastromatica |
- |
- |
+ |
- |
+ |
+ |
Sargassum cinereum* |
- |
- |
+ |
+ |
+ |
+ |
Sargassum ilicifolium* |
- |
+ |
+ |
+ |
+ |
+ |
Laminaria digita |
- |
- |
+ |
+ |
+ |
+ |
Macrocystis pyrifera |
- |
- |
+ |
+ |
- |
+ |
Porphyra vietnamensis* |
+ |
+ |
- |
- |
- |
+ |
Amphiroa fragillissima* |
+ |
- |
- |
- |
- |
- |
Jania adhaerens* |
- |
- |
- |
+ |
- |
- |
Gracillaria corticata* |
+ |
+ |
+ |
- |
- |
+ |
Hypnea musciformis* |
+ |
+ |
+ |
- |
- |
+ |
Centroceros clavulatum |
+ |
- |
+ |
- |
+ |
- |
Laurencia papillosa* |
+ |
+ |
+ |
- |
- |
- |
Chondrus crispus* |
+ |
- |
+ |
- |
- |
+ |
Eucheuma uncinatum |
+ |
+ |
+ |
- |
- |
+ |
Gelidiella acerosa* |
- |
- |
+ |
- |
- |
+ |
* Seaweeds distributed along Indian Coast. |
|
Bioethanol of 40 g/L is reported from green seaweed by the glucose subunits alone, whereas other sulphated
polysaccharides such as, Ulvan is yet to be explored. In brown seaweeds, mannitol is fermented to produce 40 g/L of
bioethanol whereas, techniques for conversion of alginate sugar to ethanol is still underway. Whereas, in red
seaweeds 3,6 anhydrogalactose (composed of glucose and galactose) poses a hindrance for conversion to ethanol
(Yanagisawa et al., 2013). Higher concentration of bioethanol is obtained by conversion of all the sugars present in
the seaweed which can be achieved by developing methods appropriate to each seaweed species.
Challenges in bioethanol production
Following are the challenges to be addressed for successful bioethanol production from macroalgal biomass
- Major cost reductions need to be achieved by suitable biocatalyst and optimal processes.
- Microorganisms possessing enzymes, which have ability to convert polysaccharides to fermentable sugars needs to
be screened or constructed.
- Commercial enzymes such as amylases, cellulases and proteases are available, but they are more efficient in
depolymerizing polysaccharides from terrestrial sources. To produce, these enzymes for commercial use, microbial
bioreactors are utilized by exploiting the microalgal strains to accumulate carbohydrate and directly utilize
their enzymatic or anaerobic digestion systems to produce ethanol, resulting in a cost-effective bioethanol
production process. In order to proceed with this procedure, screening of high carbohydrate accumulating
seaweeds from natural water bodies based on their growth cycle is to be known.
- Large-scale production to be economical, needs to utilize all sugars present in macroalgal biomass to achieve
100% efficiency.
- Mannitol is a non-fermentable sugar alcohol produced from brown algae, most of the anaerobic bacteria are unable
to carry out fermentation of mannitol as there is requirement of oxygen for regeneration of NAD+ for conversion
of NADH to NADPH which is obtained from mannitol dehydrogenase during oxidation of mannitol to fructose and
NADH. A facultative anaerobic bacterium, Zymobacter palmae ferments sugar alcohols, including mannitol
from Laminaria hyperborea extracts. Pichia angophorae is also seen to consume both mannitol
and laminarin and yield ethanol. Similar investigation to be carried out for ulvan, alginate, 3,6
anhydrogalactose conversions to bioethanol.
- Bioethanol is an intermediate product obtained during digestion of organic material, and is produced by specific
microbial strains only which makes it an obvious practical constraint of keeping the microbial culture from
getting contaminated by other microbes (Horn et al., 2000b, 2000a; Nguyen et al., 2017). Hence a controlled
condition needs to be maintained.
- Setting up of decentralised biorefinery systems in coastal areas with supporting infrastructure (e.g. roads,
utilities).
- Economically feasible algal bioethanol can be turned into a reality only through breakthrough technological
innovations. Getting algae to produce bioethanol—in very large volumes, at a very low cost—is the grand
challenge that young biotech firms have to shoulder.
|