Results and Discussion
Land uses in the Aghanashini River catchment are depicted in Figure 3, and details with the accuracy of
classification are listed in the Supplementary Table S2, which reveals a decline in forest cover from 86.06% (in
1973, 71.65% evergreen and 14.41% deciduous types) to 50.78% (in 2018, 23.95% evergreen and 26.83% deciduous).
The catchment is also witnessing the expansion of the commercial monoculture plantations (22.04%) of Acacia,
Eucalyptus, and Arecanut, requiring a high quantity of water, evident from field observations of sustained water
pumping and falling groundwater levels. Agriculture is practiced in the transition zones of Sirsi, the coastal areas
of Kumta, followed by the Ghats, having patches of paddy cultivation (16.18%). Horticulture is prominent along the
valley zones. Built-up areas constitute about 4.88% of the land use and are concentrated in towns—Kumta and Sirsi.
Forest fragmentation analyses reveal a decline in interior forest cover from 66.30% (1973) to 17.76% (2018). The
non-forest area now occupies about 49.34% (2018).
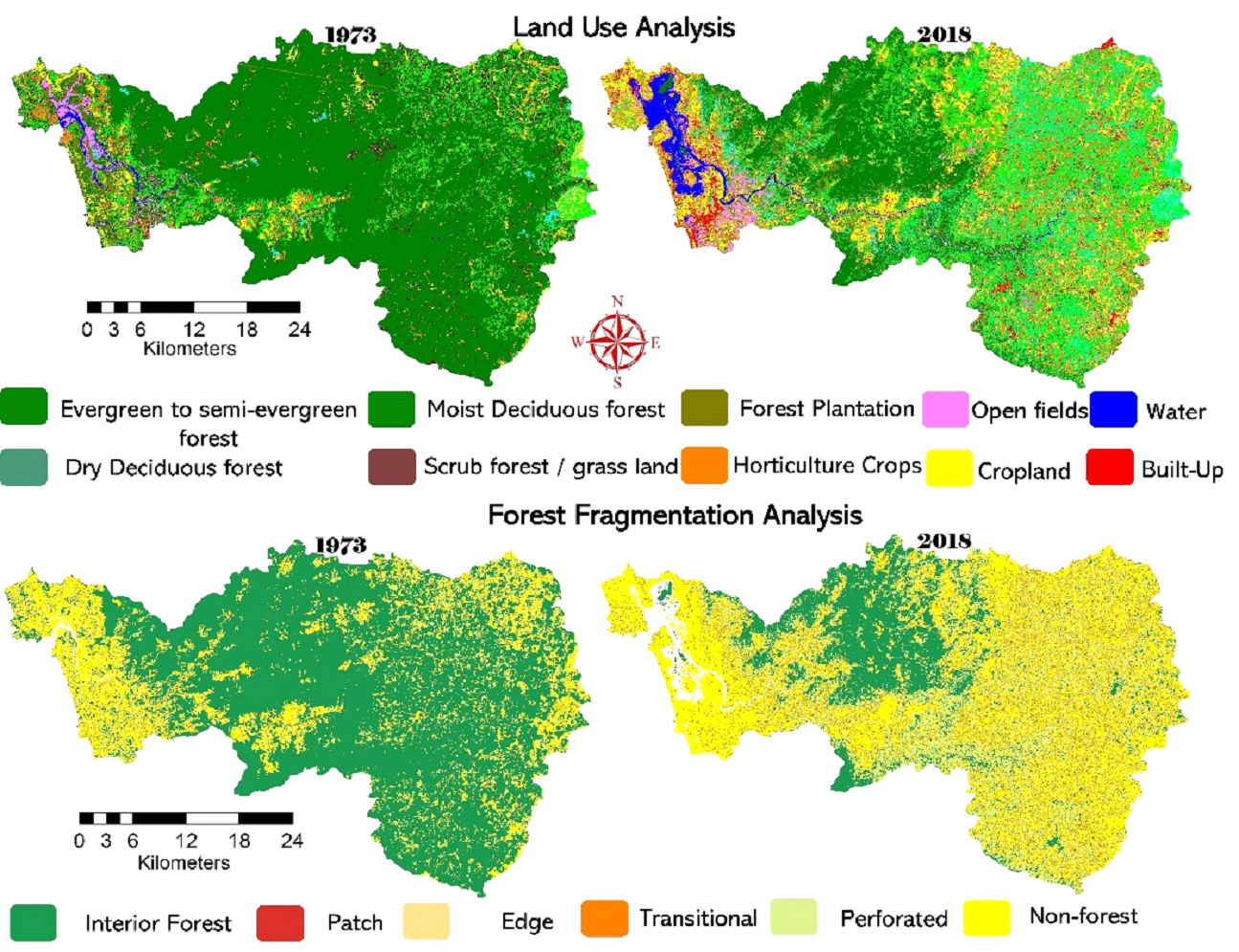
Figure 3. Land uses and extent of forest fragmentation in the Aghanashini River catchment.
3.2. Assessment of Hydrological Footprint, Ecological Footprint, and Eco-Hydrologic Footprint
Spatio-temporal analyses show that annual rainfall ranges from <3000 mm in the transition zone to >5000 mm in the Ghats and about 4000 mm in coastal zones. Figure 4 depicts the variability in rainfall across space and time. The Southwest monsoon caters to more than 80% of the total, which occurs during June and September, with the highest precipitation during the month of July.
Hydrological assessment in the Aghanashini River basin shows that gross annual rainfall in the catchment is about 3020 mm. Interception losses in the basin range between 651 mm and 1490 mm, with an average of 1042 mm. The Aghanashini River catchment has a forest cover of 60%. The run-off in the basin is about 766 mm, accounting for 1164 million cubic meters, and the balance is infiltrated, i.e., over 60% of the net rainfall is infiltrated, by the recharging subsurface (vadose and saturated zones) contributing to the subsurface flows (pipe flow and baseflow) during the non-monsoon period. Infiltration in the catchment is about 2412 million cubic meters, and the subsurface flow is about 455 million cubic meters, which caters to the water demand during all months.
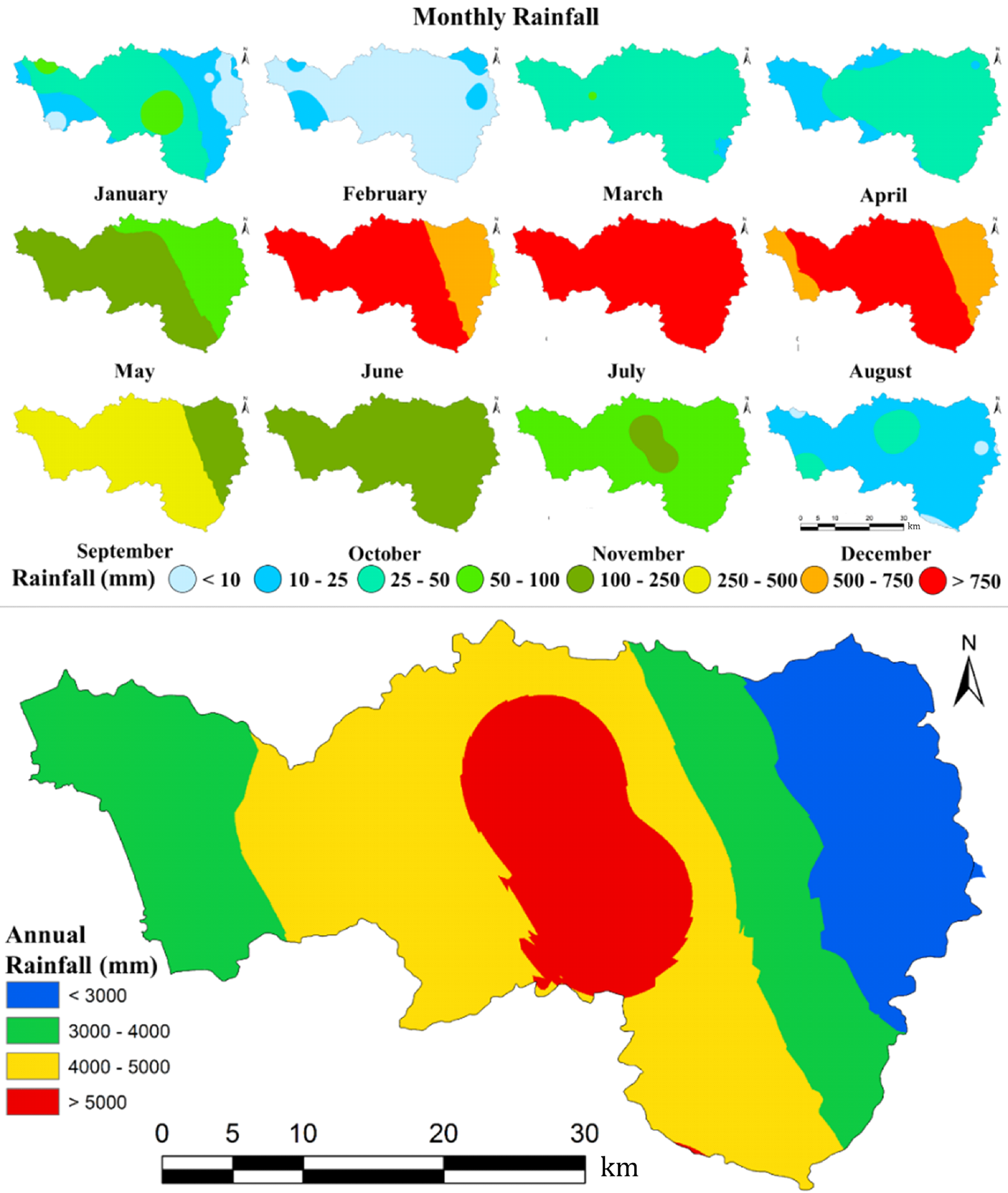
Figure 4. Spatio-temporal rainfall dynamics in the Aghanashini River basin.
The sector-wise water demand reveals that agriculture and horticulture sectors in the catchment require about 606 million cubic meter, domestic water (societal demand) is about 5.8 million cubic meter, and water required for livestock rearing is 3.8 million cubic meter. The environmental water demand includes terrestrial and aquatic ecosystems’ demand. Terrestrial water demand is the water requirements of vegetation, i.e., AET from natural vegetation (forests), and is about 937 million cubic meter. The minimum water-sustaining biota during lean seasons in the aquatic ecosystem is about 483 million cubic meter, quantified based on field investigations, which constitutes about 30% of the total flow and is comparable to similar studies in the neighboring Sharavathi, Kali, and Gangavali river basins [21,26,41,60].
The assessment of the eco-hydrological footprint at the sub-catchment level was carried out considering (i) the biotic demands of blue water demand (agriculture, domestic, livestock, and aquatic ecological needs) and green water demand (evapotranspiration) and (ii) the hydrologic regime considering the surface (overland) flow and subsurface (vadose and saturated zones) flow (pipe and baseflow) [21].
The eco-hydrological analysis sub-basin wise in the Aghanashini River catchment indicates that native forests enable a higher infiltration compared to degraded landscapes, which is explained through eco-hydrological indices (EHI), presented sub-basin wise in Supplementary Table S3. Sub-watersheds with native vegetation have a higher EHI (greater than 1), indicating higher infiltration and storage than water withdrawal due to evapotranspiration, sufficient to meet societal water requirements. The study highlights that native vegetation forests play a decisive role in retaining the water in the catchment through infiltration to sub-surface regions, which helps cater to ecological and societal demands.
The eco-hydrological footprint in the Aghanashini River basin at the sub-catchment level is illustrated in Figure 5. The hydrological footprint shows the water scarcity situation in sub-catchment 1 (in the eastern transition zones towards Sirsi town). In contrast, sub-catchments in the Ghats and Coasts (i.e., 2 to 9) show sufficient water status, catering the societal (domestic, irrigation, horticulture, and livestock) and ecological needs. The dense forest cover of native species in hilly regions (Ghats) has enhanced the water retention capability (through infiltration), which caters to the respective sub-basins’ societal and ecological water demand.
Streams were classified based on the duration of water flow and quantum as perennial (with 12 months of flow or Category A), intermittent (6–8 months of flow, Category B or C), and seasonal (4 months during the monsoon, Category D). The streams are perennial when their catchment is dominated by native species of vegetation (> 60%). This is mainly due to infiltration or percolation in the catchment as the soil is porous with native species. Diverse microorganisms interact with plant roots and the soil, which helps transfer nutrients from the soil to plants and make the soil porous [21]. Soil samples of perennial stream catchments have the highest moisture content (61.47 to 61.57%), higher nutrients (C, N, and K), and lower bulk density (0.50 to 0.57 g/cc). In comparison, soil samples from intermittent and seasonal stream catchments had higher bulk density (0.87–1.53 g/cc) and relatively lower nutrients. Figure 5 confirms the role of native forests (contiguous or interior forests) in sustaining the water, evident from the occurrence of perennial streams compared to the intermittent or seasonal streams in the catchment dominated by degraded forest patches.
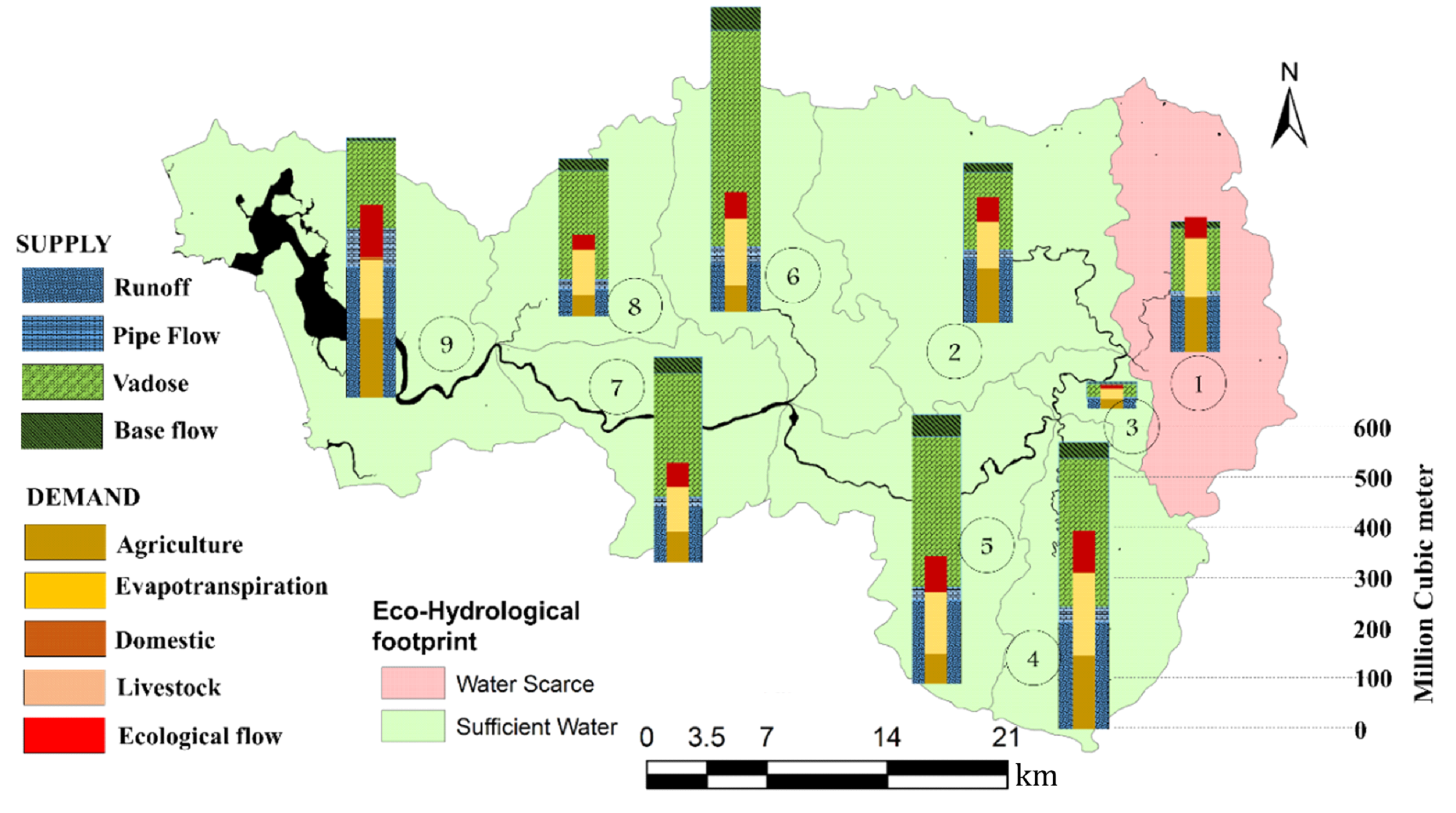
Figure 5. Eco-hydrological footprint of Aghanashini River.
Flow assessment in sub-catchments of Aghanashini indicated that forests, monoculture plantations, and agriculture played a significant role in regulating the quantum and duration of flow in streams. In sub-catchment 1 (i.e., the transition regions near Sirsi town), the catchment is dominated by monoculture plantations. The flow duration in streams is 8 to 9 months (i.e., category B). In contrast, most of the streams in other sub-catchments had water for 12 months (perennial), highlighting the role of native vegetation (Figure 6) in sustaining water throughout the year. Hence, the study emphasizes the need to maintain native vegetation cover (of > 50%) in catchments of streams and rivers to sustain water during all seasons.
Figure 6 also depicts the distribution of endemic flora and fauna (threatened categories—critically endangered, endangered, vulnerable, and near-threatened) across sub-basins in the river catchment. Biodiversity, ecology, and hydrology linkages with the land-use dynamics in sub-catchments are evident from the comparative analyses of interior forest cover, flow duration, eco-hydrological footprint, and species distribution.
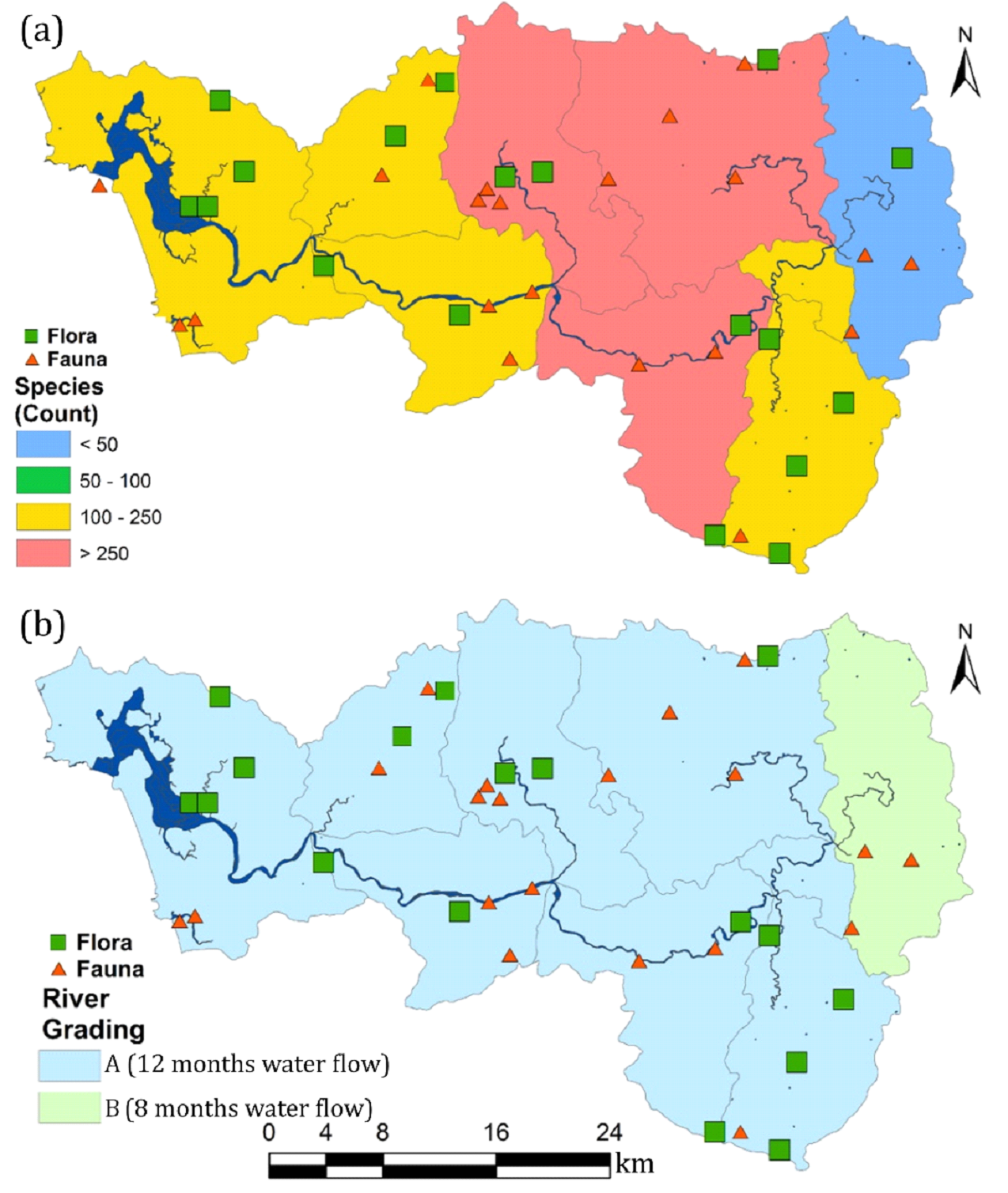
Figure 6. (a) Species distribution, (b) species distribution with
the water availability—Ecology and hydrologic regime linkages in the Aghanashini River basin.
Contiguous forests with native vegetation species have aided in sustaining the water demand (ecological and societal)
and supported diverse taxa, evident from the occurrence of rare and unique endemic and endangered taxa in the
region. The river catchment with the numerous swamps (Myristica) and endemic taxa habitats constitute the hottest
biodiversity hotspot. The current study conforms to the earlier research investigations across major rivers focusing
on diatom species and land cover dynamics in the catchment [21]. The Aghanashini River basin supports the host of
diverse epilithic diatoms: Achnanthes minutissima, Achnanthidium sp., Brachysira
neoexilis, Brachysira sp., Brachysira wygaschii, Cocconeis placentula, Cymbella
sp., Eunotia minor, Eunotia rhomboidea, Fragilaria biceps, Fragilaria ulna, Gomphonema
difformum, Gomphonemadi minutum, Gomphonema gandhii, Gomphonema parvulum, Gomphonema sp.,
Navicula cryptocephala, Navicula leptostriata, Navicula sp., Navicula symmetrica,
Planothidium frequentissimum, and Planothidium sp. [77,78]. The study highlights that the
catchment’s integrity determines the diatom species composition and water quality in the streams.
3.3. Assessment of Water Quality and Composition
The physicochemical assessment (18 parameters) reveals that the water quality parameters varied across sampling
stations during the study period. The average values of physicochemical parameters at different sampling sites are
presented in Supplementary Table S4. Yaana (YK) has the highest pH, total alkalinity, total hardness, calcium, and
magnesium among the sampled locations. In contrast, AG has the least TDS, EC, total alkalinity, pH, total hardness,
calcium, magnesium, and nitrate. Variations in pH depend on the amount of carbonate, bicarbonate, and free carbon
dioxide in the water [79]. An increase in the number of ions increases the TDS, EC, and total hardness. The increase
in total solids concentration is attributed to clay and silt particles in stream water [80]. Higher alkalinity in
water indicates higher amounts of hydroxides, carbonates, nitrates, phosphates, and sulphates [81]. Dissolved oxygen
in water bodies depends on temperature, streamflow, aeration, photosynthetic rate, and the presence of organic
matter [82,83]. Orthophosphate and nitrate are limiting nutrients that decide the productivity of freshwater
ecosystems. An increase in nitrate and phosphate occurs due to inputs from nearby agricultural fields, and an
increase in water velocity at the downstream improves water quality [84].
Pearson’s correlation coefficient (r) was computed with p-values to understand the relationship among physicochemical
parameters [85], which reveals that TDS is strongly positively correlated with EC, pH, total hardness, total
alkalinity, calcium, and magnesium (refer Supplementary Table S5). TDS increases with EC because the charged ions
(cations and anions) conduct electricity [86]. Correlation analyses reveal that (i) EC is positively correlated with
pH, total alkalinity, total hardness, calcium, and magnesium; (ii) pH is positively correlated with total
alkalinity, total hardness, calcium, and magnesium; and (iii) total alkalinity are positively correlated with total
hardness, calcium, and magnesium. Hardness is caused by cations such as calcium and magnesium and anions such as
carbonate, bicarbonate, and chloride. BOD and COD are used to assess organic matter present in both suspended and
dissolved forms in water [87].
Wide seasonal variation is observed in the physicochemical parameters across monitored locations. Parameters such as
turbidity, DO, orthophosphate, BOD, and COD were high during monsoon due to turbulence and the transport of
sediments through run-off. In contrast, the pH was alkaline in the post-monsoon season due to the photosynthetic
activities of algae. Water temperature, total alkalinity, total hardness, calcium, magnesium, and chloride were high
in the pre-monsoon with high evaporation and low water level. The varied water quality across seasons in monitored
locations is due to changes in water quantity, flow, weather, and land use in the catchment.
Figure 7 provides the season-wise WQI of monitored streams. During the monsoon season, YK, YNK, and MH showed poor
water quality [87–89]. Other sites such as BGT, BE, AGT2, AG, AGT1, and HA showed good water quality [90–92].
Monitoring sites YK, YNK, BGT, and AGT2 showed a water quality status unsuitable for drinking [92,93], whereas sites
such as BE, AGT1, and MH showed inferior water quality in the post-monsoon season [87,92]. YK reflected a water
quality status unfit for drinking [94,95]; BE and BGT had poor water quality [95,96]; and YNK and AGT2 had inferior
water quality during the pre-monsoon season [97,98]. Increased pollutants, reduced river flow, and agricultural
run-off govern stream water quality [97,99,100].
Figure 7. WQI of monitored streams during pre-monsoon, monsoon, and post-monsoon seasons.
Cluster analysis (CA) grouped sampling sites based on the similarity of water quality [101,102]. Hierarchical cluster
analysis [103–105] yielded a dendrogram (Figure 8) that grouped nine sampling sites into three clusters (G1, G2, and
G3) based on the similarity of their physicochemical characteristics. Here, G1 has less polluted stations such as
YNK, BGT, AGT2, AGT1, and MH, with a lower quantum of all parameters except sodium. G2 has moderately polluted
stations such as BE, AG, and HA with higher physicochemical parameters (such as WT, turbidity, COD, BOD, chloride,
orthophosphate, and potassium), and discharge. Station G3 (YK) has higher values for physicochemical parameters
(such as EC, TDS, pH, total alkalinity, total hardness, calcium, magnesium, DO, and nitrate) due to the inherent
catchment properties. Yaana [YK] is a tourist location prone to unregulated anthropogenic activities and waste
(liquid and solid) mismanagement.
PCA was performed considering 17 variables, which include (i) physicochemical parameters, (ii) land use (Built-up:
BU; Evergreen Forest: EF; Deciduous Forest: DF; Forest Plantation: FP; Horticulture: Horti and Agriculture: Agri),
and (iii) catchment characteristics (Flow Duration: FlowD) (Supplementary Table S6). The PCA yielded four principal
components, which accounted for 90.68% of the total variance. The first component, PC1 explained about 49.75% of the
total variance and had positive loading on EC, pH, DO, total alkalinity, total hardness, evergreen forest, and flow
duration (Table S6), whereas there was negative loading on the deciduous forest. The decomposition of litter and the
leaching of ionic contents (Na+, K+, Mg2+, Ca2+, and Cl-) in
forested watersheds alter the stream water quality [106]. These ions increase the EC, hardness, alkalinity, and pH
of water, which favors algae growth and eventually increases DO levels. The second component, PC2 explains about
23.05% of the total variance and has positive loadings on nitrate, built up, orthophosphate, and turbidity, whereas
it has negative loadings on forest plantation. This is attributed to pollution from domestic sewage that increased
the nutrient levels. The third component, PC3, was responsible for 11.35% of the total variance and had positive
loadings on agriculture, horticulture, BOD, and COD. This factor represented pollution from untreated/raw non-point
domestic discharge that increased organic matter levels. The fourth factor, PC4, was responsible for 6.54% of the
total variance and had positive loadings on agriculture, orthophosphate, and DO. This factor highlights pollution
due to agricultural run-off. The input of pesticides and synthetic fertilizers from agricultural fields altered the
chemical integrity of pristine water resources, affecting the hydrology and ecology [66]. The land-use changes with
enhanced anthropogenic activities have altered run-off patterns and flow regimes [107–109]. Vegetative cover,
topography, slope, and quantum of rainfall in a catchment decide flow in streams [108,110–114], evident from the
increased annual surface run-off of 45 ± 14% with the conversion of forest landscape to other land uses (decline of
forests by 49.34% with an increase in built-up, and open area).
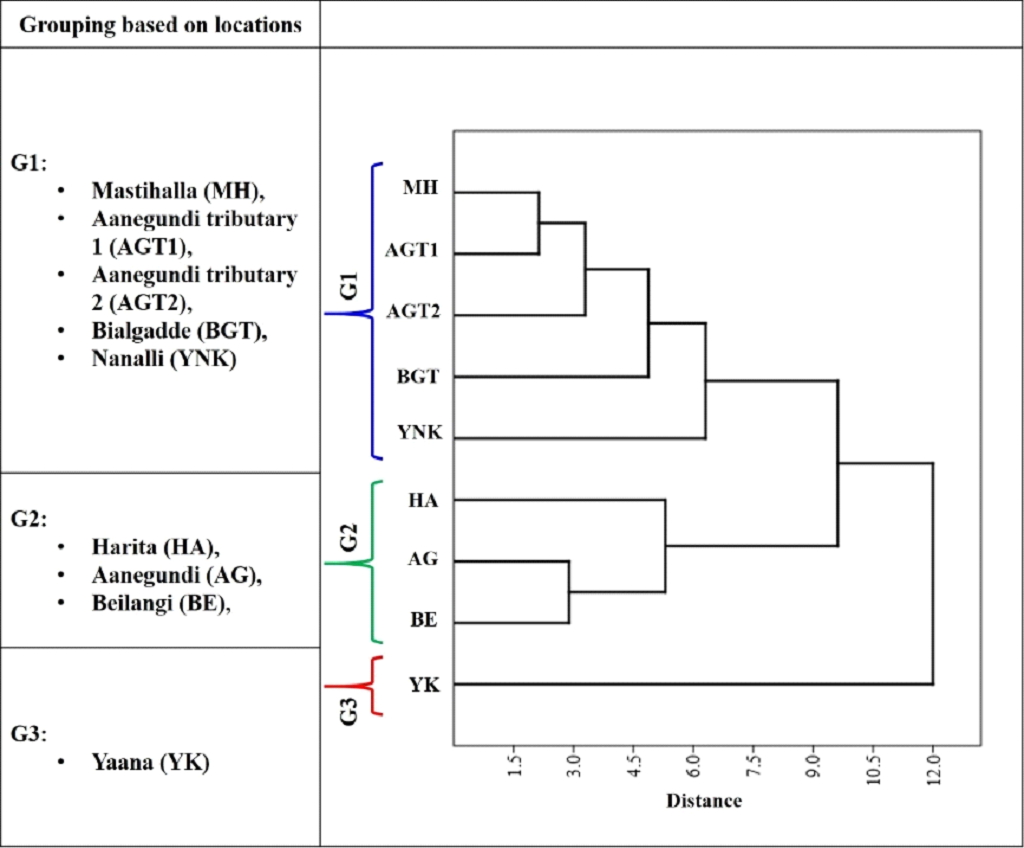
Figure 8. Dendrogram highlighting grouping of monitored streams based on physicochemical
characteristics.