Results and Discussion
3.1 Scope for bioethanol production from macroalgal biomass
The availability of spectrum of species amenable for biofuel production is one of the unique aspect of algae when
compared to other advanced feedstock [35]. Production of different types of biofuels
(bioethanol, diesel, bio-oil, and bio-hydrogen) is optimized by prioritizing species.
Characteristics of prioritized macroalgal feedstock for sustainable biofuel production are (i) availability
throughout the year (during all seasons) and (ii) presence of higher quantum of sugar.
3.1.1 Macroalgal feedstock availability and selection
Indian coast has about 1153 marine algal species belonging to 271 genera, of which 60 species are commercially
important. Rocky beaches, mudflats, estuaries, coral reefs and lagoons are the preferred habitats for macroalgae.
Indian coast harbors predominantly intertidal and subtidal algal communities [143]. Macroalgal
feedstock (for bioethanol production) distribution along the Indian Coast and islands were compiled from various
secondary data sources [144–150] along with the locations based on the current field
investigations is represented in Fig.4. Seaweed species belonging to Laminaria genera
(Brown algae or Phaeophyta) are not recorded in India and these are algae of cold waters and certain brown algae of
orders Dictyotales and Fucales (e.g. Sargassum) are distinctly warm-water plants [151] are abundantly spread and are recorded from Indian Coast. Kappaphycus
alvarezii, a red algae is native of Indonesia and Philippines and introduced in India for commercial
cultivation purpose [152], which has now colonized in various parts of Gulf of Mannar.
Fig. 4. Distribution of potential macroalgal feedstock of Indian coast
|
Setting up of bio refinery necessitates easier cultivation strategies apart from ensuring the availability of
feedstock during all seasons. During the current field investigations covering 24 months, 25 seaweed species
belonging to 19 genera were recorded from intertidal zone. Among these, eight seaweed species abundantly grows
during post-monsoon season in the Central West Coast. These species include Enteromorpha intestinalis (938.5
g/m2), Ulva lactuca (1024.5 g/m2), Chaetomorpha media (441.8 g/m2),
Gracilaria corticata (1039.5 g/m2), Gelidium pusillum (205.4 g/m2),
Grateloupia lithophila (196.6 g/m2), Sargassum ilcifolium (1175.7
g/m2) and Padina tetrastromatica (1506.7 g/m2), which were considered for
further analysis of biochemical composition (Fig. 5). It was seen that green seaweeds; U.lactuca and
E.intestinalis, and red seaweed G.corticata occurred in sufficient quantity during all seasons.
Occurrence of Ulva and Enteromorpha in all the seasons is attributed to their euryhaline nature,
whereas G.corticata species are strictly marine and are restricted to open ocean environment [153]. Year round optimal biomass production of these macroalgal species, overcomes the
seasonal constraints faced by the first and second generation biofuel feedstock [45].
3.1.2 Biochemical Composition of selected seaweeds
Macroalgal species with the higher quantum of sugar plays an important role as the composition of sugar influences
the ethanol yield. Biochemical composition of chosen macroalgae were carried out focusing mainly on higher
carbohydrate content which is an essential parameter for prioritizing feedstock for bioethanol production (Table 5). Algal biomass are composed of large quantities of carbohydrates in the cell
wall mostly in the form of structural (cellulose) and storage (starch) polysaccharides, that serve as substrate for
fermentation. Highest total carbohydrate was recorded from green seaweed Ulva lactuca (62.15±12.8 %)
followed by G. lithophila (60.5±11.4%), G.pusilum (50.1±3.4%) and E.intestinalis
(40.1±14.6%). Among these, G.lithophila and G.pusilum have lower biomass production as compared to
Ulva and Enteromorpha, which makes them unsuitable as feedstock for biofuel production. Protein
ranged from 3.7±0.94 to 27.3±15.21%. Highest protein content was recorded from C. media (27.3±15.21%)
followed by E. intestinalis (20.4±0.67%) and U. lactuca (17.3±1.68%). In green seaweed Ulva,
protein concentration ranged from 9 to 33%. Lowest protein recorded was from G. lithophila (3.7±0.94%). In
general, protein fraction is lower in brown seaweeds (3-15% DW) compared to green or red seaweeds (10-47% DW)
similar to the earlier report [154]. Lipid ranged from 0.5±0.2 to 6±4.4%. Highest lipid was
estimated in U. lactuca (6±4.4%) followed by G. pusillum (3.7±1.4%), P. tetrastromatica (2.8±0.3%).
Lowest lipid content estimated in G. corticata (0.5±0.2%). Transesterification of lipids extracted from
Enteromorpha biomass yielded 90.6% biodiesel [135], which highlights that Enteromorpha
as a promising feedstock for biodiesel production.
Elemental analysis of the seaweeds indicates 25.31-37.95% of carbon, 4.52-6.48% hydrogen and 1.88-4.36% Nitrogen.
Highest carbon, hydrogen and nitrogen content were recorded respectively from G.pusillum (C: 37.95%),
G.pusillum (H: 6.48%) and E.intestinalis (N: 4.36%) respectively. Higher cellulose content was
estimated in U.lactuca (14.03±0.14%), followed by E.intestinalis (12.10±0.53%) and
C.media (10.53±0.17%) least cellulose content was estimated in G. corticata (0.87±0.07%).
Cellulose is a glucan present in green seaweeds, which can be easily hydrolysed by using enzyme and subsequently
fermented to produce bioethanol. Green seaweeds are rich in cellulose content (>10%) [37,89,105]
when compared to red and brown seaweed (2-10%).
3.1.3 Reducing sugar extraction using pretreatment methods
Chosen seaweeds composed of all the three types of algae (green, red and brown) with varied amounts of sugar and the
complexity of the seaweed is reflected between structural and carbohydrate components [105,155,156].
It is therefore important to carefully choose the pretreatment process based on the biomass and an appropriate
efficient pretreatment process to achieve a high yield of sugar for low energy input. The reducing sugar was
determined using various pretreatment method as shown in Fig. 5. Pretreatment using boiling
water yielded very low reducing sugar and it was observed that red and brown algae formed gel during this
pretreatment process due to the presence of sugars like Agar, Carrageenan and Alginate which possess hydrocolloid
properties [70,157,158]. It is seen that, liquid hot water pretreatment releases most of
oligosaccharides [159]. U. lactuca biomass was subjected to four different
pretreatments namely; ethanol organ solvent, alkaline, liquid hot water and ionic liquid treatments. Organo solvent
and liquid hot water treatment produced highest glucan recovery of 80.8 g/100g DW and 62.9 g/100g DW respectively [160]. Sonication pretreatment also yielded lower reducing sugar, since sonication is
most commonly used in extraction of lipid from microalgae [161], highest fatty acid
composition of C16:0 and C18:1 was achieved in Spirogyra sp. employing sonication as cell disruptions
technique [40]. Higher reducing sugar was extracted from all types of seaweeds during the
dilute acid pretreatment. Dilute acid reduces the degree of polymerization resulting in recovery of 80-90% of
hemicellulose exposing cellulosic fraction accessible to enzyme digestion [162]. Pre-treated
Palmaria palmata, a red alga was exposed to acid hydrolysis, generated 218 mg/g of reducing sugar [133] and Grateloupia lithophila gave 191 mg/g of reducing sugar in the current
study. Hence, it is feasible to obtain fermentable sugars with lower inhibitor concentration using
extremely lower acid concentration [163]. Benefits of treating biomass with extremely low acid
concentration simplifies downstream process such as neutralization and waste treatment. Reducing sugar of 14.7 g/L
was obtained from E.intestinalis by dilute acid concentration of
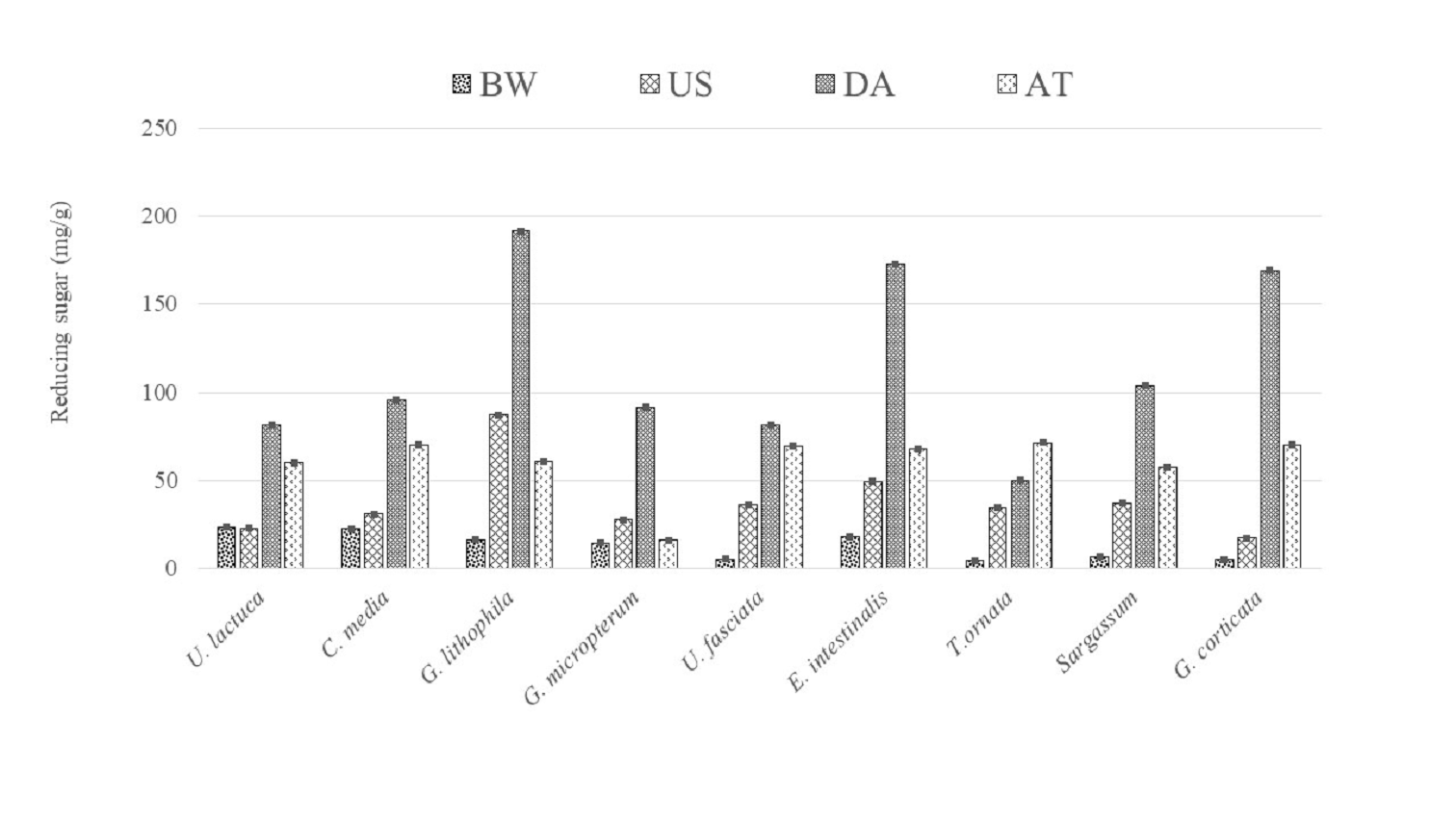
Fig. 5. Reducing sugar (mgg
-1) extracted using various
pretreatment methods for selected seaweeds
Table 5
Biochemical composition (%) of seaweeds along Central West Coast of India |
Seaweeds |
Total carbohydrate |
Soluble carbohydrate |
Insoluble carbohydrate |
Protein |
Lipid |
Cellulose |
C |
H |
N |
U.lactuca |
62.15±12.8 |
5.5±0.07 |
56.5±12.7 |
17.3±1.68 |
6±4.4 |
14.03±0.14 |
25.31 |
5.44 |
2.61 |
E.intestinalis |
40.1±14.6 |
7.5±0.02 |
32.5±14.5 |
20.4±0.67 |
2.8±0.1 |
12.10±0.53 |
33.00 |
6.44 |
4.36 |
C.media |
25.5±11.2 |
5.3±0.02 |
20.1±11 |
27.3±15.21 |
0.6±0.01 |
10.53±0.17 |
30.14 |
5.31 |
3.28 |
G.corticata |
28.2±11.1 |
13.4±0.01 |
14.7±11 |
14.4±1.33 |
0.5±0.2 |
0.87±0.07 |
26.46 |
5.01 |
1.89 |
G. pusillum |
50.1±3.4 |
4.7±0.01 |
45.4±3.4 |
8±2.04 |
3.7±1.4 |
1.55±0.05 |
37.95 |
6.48 |
3.45 |
G. lithophila |
60.5±11.4 |
14.8±0.01 |
45.7±11 |
3.7±0.94 |
2.4±1.3 |
6.23±0.16 |
29.60 |
6.15 |
2.52 |
S. ilcifolium |
26.4±13.4 |
3.6±0.01 |
22.7±13 |
12.4±0.67 |
1.9±0.01 |
1.30±0.09 |
26.20 |
4.52 |
1.88 |
P.tetrastromatica |
32.5±13.23 |
3.5±0.02 |
28.9±13.2 |
9.7±1.76 |
2.8±0.3 |
1.48±0.07 |
30.68 |
5.54 |
2.35 |
75 mM H
2SO
4 [120]. Sequential acid hydrolysis was carried out to concentrate the sugar:
hydrolysate of first cycle was utilized as hydrolyzing liquid for the 2
nd cycle and up to 5
th
cycle
[117] and generated 72 g/L of reducing sugar at the end of 5
th cycle during
0.9N H
2SO
4 hydrolysis from the seaweed granules. However, loss of liquid volume encountered in
the hydrolysate that is attributed to the sorption loss by residue. Pulverized wet biomass of
Gelidium
amansii, red seaweed was subjected to continuous acid hydrolysis which yielded higher galactose and lower
inhibitor concentration than batch reactor
[126].
Utilization of red and brown seaweeds biomass for bioethanol production can lead to debate on hydrocolloid versus
fuel affecting the existing multibillion hydrocolloid industry [89]. Therefore, for further
processes of detoxification, enzyme hydrolysis and fermentation, two seaweeds Ulva lactuca and Enteromorpha
intestinalis were selected as both the species satisfy the criteria of potential feedstock for bioethanol
production such as; annual availability, carbohydrate rich biomass, producing higher reducing sugar concentration,
ease of harvest by mechanical means, amenable to transplanting and reproducing prolifically in given environment [89].
3.1.3.1 Detoxification of acid hydrolysate
Detoxification of inhibitors (HMF and LA) resulting from acid hydrolysis was carried out using activated charcoal [164], which removed 38.8% LA and 70.37% HMF. However activated charcoal also removed sugars
14.5% glucose and 20.3% galactose present in the hydrolysate [141]. In the current study,
highest sugar removal of 63.5% and 52% was recorded from activated charcoal for U.lactuca and
E.intestinalis acid hydrolysate respectively. Similarly, Ca(OH)2 was used for neutralizing the
hydrolysate, which led to gypsum (calcium sulphate) formation, which was removed through filtration [117] also calcium ions catalyzes alkaline degradation of mono-saccharides in the over
liming process, resulting in removal of fermentable sugars [141]. Similar results were
observed in the current study, where Ca (OH)2 treatment removed sugar from
U.lactuca (56.1%) and E.intestinalis (23.3%) acid hydrolysate. Least effect of sugar removal was
recorded in Na2CO3 neutralization process (Table 6) U.lactuca (39.8%)
and E.intestinalis (14.7%).
3.2 Enzyme hydrolysis
Enzyme hydrolysis of U.lactuca and E.intestinalis yielded lower reducing sugar, compared to
pretreated biomass during the incubation period. In order to overcome recalcitrance in second generation feedstock,
the biomass was pretreated prior to enzyme hydrolysis. The pretreatment removed the lignin and hemicellulose
exposing the cellulose fibers for enzyme hydrolysis [64,66,99,165]. Pretreated biomass is then
subjected to enzyme hydrolysis, where cellulase enzymes bind to cellulose and disintegrate it into simple
sugars (glucose). Algal biomass in the current study was pretreated using dilute acid and then subjected to enzyme
hydrolysis (Fig. 6a and 6b). Pre-treatment breaks down the crystalline
cellulose structure, the major constituents in the biomass cell walls and maximize enzymatic conversion to reducing
sugars [53,105,155,166]. Despite longer incubation period for sugar release, enzyme hydrolysis
is widely preferred due to the absence of any inhibitors formation [70].
3.3 Bioethanol production from selected macroalgae
Production of bioethanol from carbohydrate rich biomass is economical and sustainable. However, investigations are
focused on improving the yield of bioethanol from seaweeds using appropriate microorganisms that have capabilities
to convert all sugars present in the seaweeds and achieve ethanol yield of 0.47 g/g reducing sugar [89,167,168]. SHF and SSF process was carried out for E.intestinalis and U.lactuca
feedstock.
3.3.1 SHF and SSF
Macroalgal feedstock E.intestinalis (EI) and U.lactuca (UL) were pretreated using 0.7 N
and 0.5 N H2SO4 respectively. Seaweed hydrolysate was neutralized and then subjected to
fermentation using CY and TY strain and conversion efficiency calculated assuming the sugar available in the
hydrolysate are fermentable (Table 7). Higher ethanol yield of 1.63 g and 25.8% efficiency was
recorded for EITY combination, whereas ULCY produced lower ethanol yield of 0.37 g achieving 12.1% efficiency.
Co-fermentation of E.intestinalis hydrolysate yielded 0.8g with 21.7% efficiency, whereas
U.lactuca yielded 0.63 g/g achieving 20.4% efficiency [120]. Pretreated E.intestinalis
to 75 mM H2SO4 and subjected to SHF and obtained ethanol of 8.6 g/L (0.86 g) achieving 30.5%
efficiency at 48 h. At the end of fermentation 10 g/L of reducing sugar remained unutilized indicating presence of
non-fermentable sugar not consumed by the yeast S.cerevisiae. U.fasciata enzyme hydrolysate was subjected
to fermentation and 1.28 g of ethanol yield was produced achieving 88.27% efficiency indicating higher reducing
sugar conversion efficiency and ethanol yield than those reported for red and brown seaweeds [169].
SSF of E.intestinalis produced 7.6 g/L with 26.9% efficiency, lower yield was attributed to the suboptimal
temperature of 32oC which is unsuitable for enzyme hydrolysis [120]. In this study,
SSF process (Table 8) produced higher efficiency when compared to SHF.
Table 6
Comparison of reducing sugar removal after neutralization process |
|
Ulva lactuca |
Enteromorpha intestinalis |
Before treatment (mg/g) |
After treatment
(mg/g) |
% Sugar removal |
Before treatment
(mg/g) |
After treatment
(mg/g) |
% Sugar removal |
Na2CO3 |
206.34 |
124.12 |
39.8 |
201.09 |
171.53 |
14.7 |
NaOH |
113.78 |
44.9 |
153.88 |
23.5 |
Activated Charcoal |
75.29 |
63.5 |
96.50 |
52.0 |
Ca(OH)2 |
90.51 |
56.1 |
154.18 |
23.3 |
Fig. 6a. Enzyme hydrolysis for direct biomass (DB)
and acid pretreated biomass (PB) for
E.intestinalis
Fig. 6b. Enzyme hydrolysis for direct biomass (DB)
and acid pretreated biomass (PB) for
U.lactuca
Table 7
Fermentation of macroalgal feedstock by the process of separate hydrolysis and fermentation (SHF) and
Separate hydrolysis and Co-fermentation (SHCF) |
Macroalgal feedstock |
Process |
Combinations |
Biomass (g) DW |
Initial sugar (g) |
Final sugar (g) |
Fermented sugar (g) |
Ethanol yield (g) |
Theoretical yield |
Efficiency (%) |
Enteromorpha intestinalis |
SHF |
EICY |
50 |
22.5 |
10.71 |
11.79 |
1.40 |
6.01 |
23.4 |
EITY |
10.13 |
12.37 |
1.63 |
6.31 |
25.8 |
SHCF |
EITYCY |
15.09 |
7.41 |
0.82 |
3.78 |
21.7 |
Ulva lactuca |
SHF |
ULCY |
19.2 |
13.17 |
6.03 |
0.37 |
3.07 |
12.1 |
ULTY |
12.72 |
6.48 |
0.52 |
3.31 |
15.6 |
SHCF |
ULTYCY |
13.10 |
6.10 |
0.63 |
3.11 |
20.4 |
CY: Cashew yeast; TY: Toddy yeast |
Table 8
Fermentation of macroalgal feedstock by the process of Simultaneous Saccharification and Fermentation
(SSF) and Simultaneous Saccharification and Co-fermentation |
Macroalgal feedstock |
Process |
Combination |
Biomass (g) DW |
Cellulose (g) |
Final sugar (g) |
Fermented sugar (g) |
Ethanol yield (g) |
Theoretical yield |
Efficiency (%) |
Enteromorpha intestinalis |
SSF |
EICY |
20 |
1.8 |
0.34 |
1.46 |
0.25 |
0.74 |
33.3 |
EITY |
0.56 |
1.24 |
0.49 |
0.63 |
77.4 |
SSCF |
EITYCY |
0.52 |
1.28 |
0.27 |
0.65 |
41.8 |
Ulva lactuca |
SSF |
ULCY |
1.7 |
0.54 |
1.16 |
0.33 |
0.59 |
54.9 |
ULTY |
0.54 |
1.16 |
0.39 |
0.59 |
65.5 |
SSCF |
ULTYCY |
0.66 |
1.04 |
0.60 |
0.53 |
113.0 |
CY: Cashew yeast; TY: Toddy yeast |
The combination of EITY
produced higher ethanol yield of 0.49g/g achieving 77.4% efficiency. Simultaneous saccharification and
co-fermentation (SSCF) of
E. intestinalis produced 0.27 g/g of ethanol with 41.8% efficiency whereas
U.lactuca produced efficiency of 113 % indicating fermentation of sugars other than glucan.
Candida
shehatea, Scheffersomyces stipitis (Pichia stipitis), and
Pacchysolen tannophilus are the most
promising yeast species for conversion of
Xylose
[112], which can be used in
combination with
S.cerevisiae in SSCF process to obtain higher ethanol yield.
[104]
subjected cellulosic residue of
Kappaphycus alvarezii along with galactose synthetic medium to SSCF using
single strain
S.cerevisiae CBS1782 and recorded 64.3 g/L of ethanol indicating utilization of galactose
sugar along with glucose. Co-fermentation of corn stover using
S.cerevisiae and commercial strain TMB3400
yielded 40g/L or ethanol with 59% theoretical efficiency
[74].
S.cerevisiae and
S.
stipitis were used for SSCF of Rice straw and produced 15.2g/L of ethanol
[170].
Seaweeds contain low amounts of polysaccharides composed of glucose. Production of ethanol, therefore, needs to be
from carbohydrates including sulfated polysaccharides, sugar acids and sugar alcohols. However, inability of
microorganisms in fermenting all sugars present in seaweeds into ethanol is a major drawback. Therefore, isolation
of yeast strains to ferment both pentose (C5) and hexose (C6) sugars are vital for achieving higher ethanol
yield.
However, recent studies are focusing on non-controversial cellulosic residue following extraction of hydrocolloid
from seaweed biomass [60]. But the lower cellulose content of residue prevents it from being a
viable feedstock option considering the emergent demand for bioethanol. This necessitates the selection of seaweed
species with higher cellulose content together with higher growth rate for sustainable bioethanol production [89,171]. Red algae has more agar and carrageenan and brown algae has more algin and
lower cellulose compared to green seaweeds, that have cellulose making up to 70% of their dry weight (as
Cladophorales and Ulvales) [176].
It is evident from the experimental results, that green algae are apt as potential feedstock due to higher
carbohydrate and cellulose contents. Higher growth rates have been recorded for green seaweeds ranging from
19.15-24.25% when compared to red macroalgae (3-8%) [89,177,178]. Green seaweeds have
production potential that is 2-20 times that of conventional terrestrial energy crops [179].
Red and brown seaweed’s lower cellulose content of residue and lower growth rate prevents it from being a viable
feedstock to meet the growing demand for bioethanol. Comparison of ethanol yield from other potential macroalgal
feedstock are represented in Table 9
3.3.2 Multivariate analysis of process condition for bioethanol production
Downstream process of bioethanol production from macroalgal biomass is dependent on various factors such as dilute
acid pretreatment conditions (acid concentration, temperature, incubation time), enzyme hydrolysis conditions (pH,
temperature and incubation time), reducing sugar and fermentation conditions (temperature and incubation time). In
order to understand influence of each of these factors in ethanol production, multivariate regression analysis (Table 10) was performed and the probable relationship is given by the equation 2.
Overall this model explains 97.3% variation in the data.
Table 9
Comparison of reducing sugar, ethanol yield and percent theoretical yield from various macroalgal
biomass |
Seaweed species |
Reducing sugar g/l |
Ethanol yield g/g |
%Theoretical yield |
References |
Green Seaweeds |
Chaetomorpha media |
27.79 |
0.057 |
10.15 |
* |
Ulva fasciata |
21.82 |
0.43 |
83.66 |
[89] |
Ulva lactuca |
1.12 |
0.39 |
77.03 |
[94] |
Ulva lactuca |
35.43 |
0.23 |
45.62 |
* |
Ulva pertusa |
43 |
0.43 |
84.36 |
[70] |
Ulva pertusa |
26 |
0.48 |
93.51 |
[97] |
Enteromorpha intestinalis |
48.96 |
0.25 |
49.37 |
* |
Enteromorpha intestinalis |
45.56 |
0.21 |
41.74 |
[120] |
Red Seaweeds |
Gelidium elegans |
49 |
0.38 |
73.63 |
[70] |
Gelidium amansii |
43.5 |
0.47 |
92.40 |
[108] |
42.2 |
0.38 |
74.51 |
[126] |
7.93 |
0.42 |
82.34 |
[172] |
7.93 |
0.48 |
93.46 |
Gracilaria verrucosa |
34.63 |
0.43 |
84.31 |
[60] |
19.70 |
0.43 |
84.31 |
[110] |
Gracilaria corticata |
57.90 |
0.01 |
0.98 |
* |
Gracilaria salicornia |
13.8 |
0.079 |
15.49 |
[173] |
Gracilaria sp. |
11.46 |
0.42 |
82.35 |
[132] |
Grateloupia lithophila |
44.45 |
0.09 |
17.85 |
* |
Gelidium pusillum |
36.96 |
0.04 |
8.49 |
* |
Eucheuma cottonii (Kappaphycus alvarezii) |
11 |
0.45 |
89.13 |
[88] |
81 |
0.45 |
88.24 |
[104] |
20.4 |
0.21 |
41.18 |
[164] |
51.9 |
0.42 |
82.36 |
[117] |
Palmaria palmata |
21.84 |
0.173 |
33.92 |
[133] |
Brown Seaweeds |
Alaria crassifolia |
67.20 |
0.38 |
74.40 |
[70] |
Laminaria hyperborea |
30 |
0.43 |
84.31 |
[114] |
Padina tetrastromatica |
17.9 |
0.01 |
2.53 |
* |
Sargassum sagamianum |
25.9 |
0.386 |
75.69 |
[116] |
Sargassum sagamianum |
2.55 |
0.89 |
174.55 |
[174] |
Sargassum sagamianum |
19.8 |
0.35 |
69.32 |
[125] |
Sargassum ilcifolium |
27.04 |
0.05 |
9.60 |
* |
Sargassum fluvellum |
9.6 |
|
|
[54] |
Saccharina japonica |
6.72 |
0.343 |
67.25 |
[163] |
Saccharina japonica |
34 |
0.41 |
80.74 |
[103] |
Saccharina japonica |
45.6 |
0.17 |
33.11 |
[52] |
Saccharina japonica |
75 |
0.34 |
67.45 |
[116] |
Saccharina latissima |
35 |
0.45 |
88.24 |
[36] |
Undaria pinnatifida |
42.9 |
0.22 |
43.05 |
[120] |
Undaria pinnatifida |
20 |
0.144 |
28.24 |
[107] |
Seagrass |
Zosteria marina |
92 |
0.059 |
11.5 |
[175] |
*Current work |
Table 10
Coefficients and SE values of multivariate regression analysis |
Process |
Process condition |
Coefficients |
Standard Error |
Dilute acid pretreatment |
Concentration |
-4.09 |
1.14 |
Temperature |
0.12 |
0.29 |
Incubation Time |
-0.13 |
0.67 |
Enzyme hydrolysis |
pH |
10.61* |
2.44* |
Temperature |
-0.31 |
0.16 |
Incubation Time |
-0.04 |
0.02 |
|
Reducing sugar |
0.32* |
0.04* |
Fermentation process |
Temperature |
-1.23 |
2.09 |
Incubation Time |
-0.12* |
0.02* |
*p<0.05 |
Y=-2.75- 4.09 (X1) + 0.12 (X2) - 0.13 (X3) + 10.61 (X4) - 0.31
(X5) - 0.04 (X6) + 0.32 (X7) - 1.23 (X8) - 0.12 (X9)………………..
(Eq. 2)
Where,
Y: Ethanol g/L, X1: Acid concentration (mM), X2: Acid hydrolysis Temperature (oC), X3:
Acid hydrolysis incubation time (min), X4: buffer pH, X5: Enzyme hydrolysis temperature (oC),
X6: Enzyme hydrolysis incubation time (h), X7: Reducing sugar in (g/L), X8:
Fermentation temperature (oC), X9: Fermentation time (h)
This analysis highlights that process conditions such as; acid pretreatment temperature, buffer pH and reducing
sugar concentration affects the ethanol yield (Eq. 2). The increase in hydrolysis temperature
during acid catalyzed reaction would also enhance the sugar production and decomposition [180,181]. Pretreatment temperature plays a vital role in sugar release as higher
temperatures often leads to the formation of inhibitor, which is detrimental to the fermentative microorganisms
leading to reduced ethanol yields [69,181]. Enzymes consists of ionic groups on their active
sites which varies along with the pH resulting in changes in the activity of the enzyme, its structure, reaction
rate and the product formation [182]. Therefore, pH of the reaction medium or buffer needs to
be optimum for higher enzyme activity [183,184]. Fermentation process is slower in low sugar
medium, whereas the process increases in the medium containing 15-20 g/L of sugar and remains stable up to 200 g/L.
Medium with 200 g/L to 300 g/L of sugar concentration lowers the growth of yeast microorganisms [185–187].
However, higher concentration of fermentable sugars yield higher ethanol, whereas non-fermentable sugars (pentose)
can affect the fermentation yield due to lack of transport system in yeasts.
4. Scope and opportunities for macroalgal cultivation
Macroalgal cultivation can be attempted in India by taking the advantage of 2.172 million km2 exclusive
economic zone (including Andaman and Nicobar islands) as well as abandoned aquaculture ponds. Aghanashini estuary
(Lat 14.391° to 14.585° N; Long 74.304° to 74.516° E) situated in Kumta taluk, Karnataka consists of such
aquaculture ponds called gazni, which serves as potential site (Fig. 7) for macroalgal
cultivation [134]. U.lactuca and E.intestinalis abundantly grows in this
estuary during all the season and E.intestinalis naturally occurring in gazni ponds are
mostly discarded as waste. Ulva and Enteromorpha genus possess a blade-like or filamentous
morphology, which can tolerate wide range of environmental conditions such as temperature upto 40 oC [51] and tolerate salinities in the range of 10-60 ppt [188] and
resulting in higher [135].
The spatial extent of gazani lands in Aghanashini estuary (Fig. 7) is about 2000 ha
(much of it not being used for rice cultivation now) and area of 1000 ha could be considered for algal cultivation
during the late monsoon months and subsequently, these lands may be used for shrimp culturing or utilized for other
forms of fisheries. The algal production happens indeed without any external input of nutrients, and therefore the
gaznis hold very good potential for ethanol production, prior to the commencement of fishery operations,
and before salinity rises substantially with the cessation of the rainy season. The algal harvests can be
effectively made from any gazni at biweekly intervals, two weeks’ time considered fairly good for
harvestable regrowth. It means effectively 1000 ha area are available for algal harvests every week. The lean season
for fishery (of prawns and fish mainly) alone needs to be utilized for this purpose, so that prospects for ethanol
production, by no means, could come in the way of food production. These 1000 ha of aquaculture areas could be
effectively available for algal harvests during the off-season, which might benefit about 2500 small farmers, who
were once rice cultivators, who had permitted their erstwhile fields to be converted into shrimp farms, which are
not in suitable condition for reintroduction of rice without making substantial structural changes. These
gazni lands also exhibit positive correlation with water temperature, air and salinity which influences the
growth of diatoms such as Pleurosigma balticum, Melosira sp., Nitzschia sigma and Nitzchia spp.
that are potential candidates for biodiesel production [189].
Fig. 7. Potential sites for macroalgal cultivation in Aghanashini
Estuary |
Cultivation of Ulva and Enteromorpha is estimated to produce annual biomass of 45 t/ha [179] and 100 t/ha [51] respectively, which is 2-20 times the production
potential of first and second generation feedstock and 3 times the production of brown algae in temperate waters [179]. Commercial cultivation of these seaweeds largely focuses on high-value food
(aonori) and aquaculture feed production. Ammonium are abundantly present in aquaculture effluent, which is readily
assimilated by Enteromorpha and Ulva in the range of 50-90 µmol N/g DW h, which makes it feasible
to cultivate these algae in aquaculture effluents qualifying as species for bioremediation process [190,191]. However, sporadic sporulation of Ulva leads to loss of biomass due to
which attempts of cultivating at large scale has failed, also environmental factors responsible for these
sporulation have not yet been completely explored [190]. This can be overcome by technique [135], involving artificial seeding under controlled conditions. This allows control over
higher seeding density and consequently biomass production. Seeding density of 621,000 swarmers/m and nursery period
of five days were quantified as key factors affecting the growth and biomass yield of Ulva sp. This study
also highlights the shorter nursery period and culture cycles required for green seaweeds when compared to red and
brown seaweeds.
Large-scale cultivation of macroalgae in open ocean eliminates the need for external inputs such as CO2 cylinders,
monitoring of temperature or pH alteration. However in controlled conditions, all these parameters play a crucial
role in sustaining biomass productivity. Algae acquire carbon in the form of CO2 or bicarbonates, at pH
8, seawater has alkalinity of 2.3 meq which allows only 0.5 mM of CO2 or 6 mg/l of C to be absorbed from
the seawater. In order to increase the level of CO2 absorption pH needs to be increased to nearly 9 which
is not feasible as it slows down the photosynthesis [192].In a study [193], brown seaweeds (Sargassum sp.) were cultivated at different pH and
temperature and was observed that higher biomass productivity obtained at ambient pH of 7-8.2, indicating that low
pH values affected the growth of the algae.
The seaweed industry in India is still at infancy, functioning more like a cottage industry. Seaweeds are collected
from the natural stock mainly for the production of agar without any regulations [194].
Macroalgal biomass in addition to being potential feedstock for bioethanol production, also serves as a good food
supplement. Enteromorpha was tested as nutritional supplement in preparation of Indian snacks, which
resulted in higher iron and calcium content along with increase in proteins, vitamins and dietary fibres [195]. Macroalgal protein, dietary fibres and phytochemicals are utilized in
order to enhance the nutritional quality of the food products. Complete utilization of macroalgal biomass into
producing biofuel and value added products has potential to ensure India’s energy and food security [134]. The study presents the scope to utilize macroalgal biomass in producing value
added products in addition to bioethanol production, bringing resilience to the Industry and improving their
economics and usefulness. Prospects of bioethanol with the value added products would support the livelihood of
rural population, while aiding as the potential feedstock for biorefinery [36,196].