RESULTS AND DISCUSSION
3.1. Physiochemical parameter analysis
Municipal solid waste composition, elapsed time, temperature,
moisture and available oxygen are some of the important factors,
influencing the leachate quality. The leachate quality with similar
waste types may be different in landfills located in varied climatic
regions. Furthermore operational practices in landfills also influence the leachate quality. The results of physico-chemical characteristics of the leachate and samples from water bodies in
Mavallipura landfill are presented in Table 1.

3.1.1. pH
pH values of leachate (L1, L2 and L3) of the landfill site were 7.4,
7.6, 7.5 and the pH values of the P4 (pond) and G5 (open well) water
samples found to be 8.4 and 7.5. The critical reaction in MSW is the
degradation of organic materials to produce carbon dioxide and a
small amount of ammonia that further results in the formation of
ammonium ions and carbonic acid. The carbonic acid dissociates
with ease to produce hydrogen cations and bicarbonate anions,
which influence the level of pH of the system. Additionally, leachate
pH is also influenced by the partial pressure of the generated carbon dioxide gas that in contact with the leachate. Dissolved materials and gases shift the pH of natural water either to acidic or
alkaline side. pH lower than 7 are usually softer waters and the
acidity is due to carbonic, humic, fulvic and other organic acids
(Mahapatra et al., 2011a,c). pH above 7 can carry a greater load of
dissolved substances and are capable of supporting a good plant
life. The alkaline nature of leachate is an indicator of the mature
stage of the dumping site (Jorstad and Acworth, 2004).
3.1.2. Alkalinity
Alkalinity is caused by bicarbonate, carbonate and hydroxyl
ions. For landfill leachate, total alkalinity values are often found to
be significantly higher. This is because of the biochemical decomposition, and dissolution process occurring within landfill and
disposal sites. The biodegradation processes of organic matter
produces significant amount of bicarbonate, which represents
dissolved carbon dioxide which is also the major components of
alkalinity (Mahapatra, 2011b). In this investigation, the Mavallipura
leachate samples (L1, L2, L3) was found to have significantly high
alkalinity values. The high alkalinity observed in this study reflects
the level of biodegradation process taking place within the disposal
sites. The presence of significant amounts of ash and slag from the
combustion of wood, agricultural residues can potentially increase
alkalinity in leachate greatly in Mavallipura landfill sites. High
alkalinity values observed in this study therefore imply that there a
fair chances of groundwater contamination. This might produce
unpleasant odour in the water sample that is unacceptable for
many users (Meenakumari, 2004).
3.1.3. Conductivity and total dissolved solids
These parameters are influenced by the total amount of dissolved organic and inorganic materials present in the solution,
and are used to demonstrate the degree of salinity and mineral
contents of leachate. Total mineral content further refl ects the
strength and overall pollutant load of the leachate. The salt content
in the leachate is due to the presence of potassium, sodium, chloride, nitrate, sulphate and ammonia etc. Extremely high values for
conductivity are attributable to high levels of cations and anions.
High concentrations of total dissolved solids may reduce water
clarity, which contributes to light limitation resulting in a decrease
in photosynthesis and leads to an increase in water temperature.
This affects the growth and development of the biotic components
as photosynthetic bacteria and algae. High TDS limits the growth
and may lead to the death of many aquatic organisms. Electrical
conductivity is an indicator of dissolved inorganic ions in ground
water; pond (P4) and open well (G5) showed high values (values of
< 40 0e800 mS/cm: clean ground waters) in the close vicinity of
landfill site implying possible cross contamination of the leachate
with the ground waters
3.1.4. Major anions
The level of inorganic elements present in leachate is dependent
principally on the ease of leaching inorganic constituents present in
the MSW and the stabilization process in the landfill. In this
investigation, Mavallipura landfill leachate sample was found to
have considerably high concentrations of all the major anions like
chlorides, nitrates, sulphates where concentration of chloride was
highest, while sulphate was lowest. High chloride content in the
leachate sample reflects the presence of significant amount of
soluble salts in the municipal solid waste materials of the study
area. High chloride content in Mavallipura landfill leachate sample
can be attributed to landfill dumps, sewage ingress, and domestic
effluents including animal waste disposed to the site. High concentrations of chlorides were also observed in the pond (P4) and
open well (G5) close to the landfill site. Excess of chloride in water is
usually taken as an index of pollution and considered as a tracer for
groundwater contamination (Loizidou and Kapetanios, 1993).
A high chloride content in ground water can be from pollution
sources such as domestic effluents, fertilizers, septic tanks, and
leachate (Mohr et al., 2006). High chloride content in ground water
causes diseases related of heart and kidney. Sulphate in landfill
leachate is sourced primarily from the decomposition of organic
matter, soluble waste, such as construction wastes or ash, synthetic
detergents and inert waste, such as dredged river sediments. Nitrates represent the most oxidized form of nitrogen found in the
natural system. It is often regarded as an unambiguous indicator of
domestic and agricultural pollution. In leachate sample, it is formed
primarily as a result of oxidation of ammonium to nitrite and
subsequently, to nitrates by nitrification process. The knowledge of
nitrates and phosphates is important in predicting the nutrient
status of waters as these ions are important plant nutrients which
usually appear as a result of decomposition and mineralization of
organic matter.
3.1.5. Major cations
Constituents as calcium, magnesium, sodium, and potassium are
considered to be major cations typically present in leachate.
Derived from the waste material through mass transfer processes,
the concentration of these cations in leachate is specific to the
composition of the waste mass and the prevailing phase of stabilization in the landfill (Christensen et al., 2001 ). The high concentration of sodium around the landfill indicates the impact of
leachate. The high concentration of sodium causes renal, cardiac
and circulatory diseases (Mohr et al., 2006). Despite few inputs
from agricultural activities, the high concentration of potassium
has been reported to be an indication of the leachate effect (Eillas,
1980). Calcium is one of the most common cations found in
groundwater aquifers, as it dissolves from rocks, such as limestone,
marble, calcite, dolomite, gypsum, fluorite, and apatite. Magnesium
is one of the principal cations associated with water hardness
(Harmsen, 1983). Calcium concentrations were noticeably high in
open well (G5).
Sodium and potassium are both present at considerably high
concentrations in all the samples. Sodium and potassium are not
affected significantly by microbiological activities within the
landfill site. These ions play a major role in plant physiology and are
most likely derived from vegetable residues and domestic wastes.
Increased concentration of potassium in ground water is often
considered as an indicator of leachate pollution (Christensen et al.,
2001 ). The primary source of potassium is due to weathering and
erosion of potassium bearing minerals such as feldspar and leaching of fertilizer. It can have adverse health effects from exposure to
increased potassium in drinking water. Excess potassium causes
kidney failure, heart disease, coronary artery disease, hypertensions, and diabetes. Sodium and potassium being dominant cations
are not significantly affected by microbiological activities within
the landfill site. Ammonium ions can enter the aquatic environment via municipal effluent discharges and excretion of nitrogenous wastes from animal and indirect means such as air deposition,
nitrogen fixation, and runoff from the agricultural lands. High
ammonia levels in water bodies make it difficult for aquatic organisms to sufficiently excrete the toxicant, leading to a toxic build
up in internal tissues and blood and potential death. It affects the
environmental factors such as pH and temperature, can affect
growth and development of aquatic animals. Furthermore, the accuracy of the analysis was verified by ion balance method. The
ratios of the sum of the molar concentrations of anions and cations
should be nearer to 1. From the ionic balance ratio, it is confirmed
that the ratios of molar concentrations are near to 1 supporting the
accuracy of various parameters determined.
Piper diagram helps in grouping similar cations and anions and
characterization of water types (Piper and Darrah, 1994). The Piper
diagram reveals the composition of different ions (explains ionicchemistry) in percentage and also identifies the hydrogeochemical facies. By grouping sodium (Na þ) þ potassium (K þ)
together, the major cations were displayed on the trilinear Piper
diagram (Freez and Cherry, 1979). Similarly, carbonate
(CO32 ) þ bicarbonate (HCO3) are grouped together along with
sulphates and chlorides resulting in three groups of the major anions. Central diamond shape area is a matrix transformation of the
graph of anions (sulphate þ chloride/total anions) and cations
(sodium þ potassium/total cations), which represents the total
ionic. A few conclusions can be drawn from the piper diagram of
the collected leachate samples (Fig. 1 of Supplementary material).
Firstly, it indicates a predominance of select cations as Na and K in
comparison to Ca and Mg. Secondly, bicarbonates and carbonates
are the dominant anions found in the leachate samples compared
to sulphates and chlorides. The analyzed sample can be thus categorized as the NaeHCO3 type leachate. The analysis also showed
large percentages of the samples within the CaeSO4 category followed by the NaeHCO3 type. However, anions like sulphates were
very meager in concentrations compared to other anions
3.1.7. BOD and CODThe BOD5/COD ratio indicates the age of the waste fill (Hui,
2005) and the changes of biodegradable compounds in the
leachate. Any water, having its BOD5/COD ratio more than 0.63, can
hence be considered to be quite controlled due to biological activity
(Naveen et al., 2013). The value of COD and BOD5/COD can characterize the age of the landfi ll. A comparison of the values of
COD and BOD5/COD of the present studies with the earlier study
(Hui, 2005) showed that the age of all the leachate is between 5 and
10 years. This was confirmed with the actual age of dumping of
MSW. Similar studies carried earlier (Slomczynska and
Slomezynski, 2004; Bhalla et al., 2012) showed that the physicochemical characteristics of leachate are highly variable over the
course of a landfills life. Thus, the age of the landfill has a significant
effect on leachate composition. The young leachate primarily
comprises of undecomposed organic compounds that are readily
biodegradable, giving rise to refractory compounds that accumulate with the exploitation of landfill and are resistant to biochemical
degradation. Higher organic matter in leachate samples leads to
high emissions if they are not treated, that further increases the
green house gas (GHG) foot print of the area (Ramachandra and
Mahapatra, 2015). The results of the present study were similar
to studies conducted earlier (Granet et al., 1986) that showed low
BOD5/COD (~0.1) indicative of a stabilized leachate. Unlike the
present study where the BOD5/COD values of the leachate samples
were ranging from 0.1 to 0.5. The studies conducted by (Chian and
Dewalle, 1976) reported BOD5/COD of 0.5e0.7 indicating large
amounts of biodegradable organic matter.
The BOD and COD values are relatively low in the open well
sample and also the ratio of BOD5/COD in the pond (0.097) is much
higher than that of (0.006) open well. This is mainly due to relatively high COD values in the pond. This can be possibly due to
contamination of pond with leachate from nearby MSW landfill.
Assuming that the pond (P4) sample is partially contaminated with
the leachate, the relatively lower BOD5/COD values may be due to
contribution by algae. Moreover the pond (P4) sample is characterized not only by low BOD and COD values but also by lower
BOD5/COD ratio. Prolific growth of algae in the ponds provided a
green coloured appearance to the pond water (P4). High density of
these algae can result in high photosynthetic activity thereby
generating voluminous oxygen that help in oxidation of the contaminants in the lake at the same time providing oxygen for the
heterotrophic bacteria that in turn helps in aerobic treatment of
organic matter present in these ponds (Mahapatra et al., 2011a,b,c).
The presence of algae for the production of oxygen and primary
productivity is essential for any healthy water body (Granet et al.,
1986; Mahapatra et al., 2011b). BOD5 to COD ratio revealed medium aged leachate samples (5e10 years). However earlier studies
on leachate samples showed high concentration of organic constituents that were beyond the permissible limits (Ehrig, 1989).
3.2. Heavy metal and elemental analysis of solidsThe metal analysis showed high concentrations of iron in the
leachate, followed by zinc, and nickel. The concentrations of chromium, copper, cadmium and lead were low. These trace elements
are considered to be dangerous pollutant. In a living system they
are capable of disrupting normal functions of a cell by virtue of their
capacity to form strong metallic bonds with a number of functional
macromolecules at the same time causing clump formation. Minute
concentrations of chromium can cause nausea and vomiting and is
also toxic to crops. Lead causes anemia, brain damage, anorexia,
mental deficiency, vomiting and even death in human beings
(Maddock and Taylor, 1977; Bulut et al., 2006) and is toxic even at
lower concentrations. Cadmium has been reported to cause
agonistic and antagonistic effects on hormones and enzymes
leading to lots of malformations like renal damage (Lewis, 1991;
Donalson, 1980) and are toxic at low concentrations also (Kale
et al., 2010). Both cadmium and lead have been classified as carcinogens (USEPA, 1999). Other trace metals, such as Ni, Zn, Cu have
also been reported for various health problems with possibility of
bio-accumulation in the food web (Langston, 1990).
The oven dried leachate solids showed the presence of trace
metals as Hg, Sn, Cr, Ni, Zn, Co and Fe as shown in the SEM-EDXA
analysis. High S percentage (~8%) at a low redox value indicate
the possible formation of metal sulphides. Leachate ponds at
anaerobic conditions with a higher quantum of sulphates with the
availability of organic C promote the growth of sulphate reducing
bacteria. Most of the heavy metals react with hydrogen sulphide
and leads to the formation of highly insoluble metal sulphides
(Mahapatra, 2015). Bacterial sulphate reduction results in the precipitation of dissolved metals as metal sulphide solids. Other trace
metals as copper, lead, zinc, cadmium, etc., also form highly
insoluble sulphide compounds in contact with the low concentration of hydrogen sulphide. Most of the trace elements are readily
fixed as sulphides and get accumulated in soils, and because this
process is largely irreversible, repeated applications of amounts
more than plant needs eventually results in soil contamination
rendering it non-productive (Ahmed, 2012).
Heavy metals often get removed from the aqueous leachate
phase through physical forces of settling, flocculation and sedimentation attributed to the specific gravity of the particulate
matter (ITRC, 2003). Flocculation is enhanced by high pH, suspended matter, ionic strength and by the presence of algal groups
(Matagi et al., 1998). Apart from the physical processes, the
chemical removal processes mostly the adsorption, oxidation and
hydrolysis of metals, precipitation and co-precipitation play a
critical role in concentrating heavy metals. During sedimentation,
heavy metals are adsorbed to the soil particles by either cation
exchange or chemisorption. Heavy metals are mostly adsorbed to
the clay and organic matter present in the leachate by electrostatic
attraction (Patrick and Verloo., 1998).
The SEM EDXA analysis showed the presence of clay-like substances as they comprise of Aluminium phyllosilicates with Al
(~6%), Si (~8%) and O (~36%) that can help in metal trapping through
cation exchange capacity. The total capacity of a soil for retaining or
holding exchangeable cations is called cation exchange capacity
(CEC). CEC influences the soil's ability to hold onto essential nutrients and provides a buffer against soil acidification. CEC increases
with certain substrates with increasing clay and organic matter
content. Cation exchange involves the physical attachment of cations (positively charged ions) to the surfaces of clay and organic
matter by electrostatic attraction. However, chemisorption represents a stronger and permanent form of bonding than cation exchange. High incidence of Fe in leachate also indicates the
formation of insoluble compounds through hydrolysis and oxidation that can occur in leachate ponds. This leads to the formation of
a variety of oxides, oxo-hydroxides, and hydroxides (Woulds and
Ngwenya, 2004). Iron removal depends on pH, oxidationereduction potential and the presence of various anions (ITRC,
2003).
Precipitation depends on the solubility product Ksp of the metal
involved, pH of the redox environment and concentration of metal
ions and relevant anions. In this study precipitation from a saturated solution of a sparingly soluble heavy metal salt could have
taken place at the low redox conditions. Similarly there are ample
chances of co-precipitation which is also an adsorptive phenomenon in rapidly settling systems largely in the presence of Fe where
usually heavy metal co-precipitates with secondary minerals in
leachate ponds. Metals as Cu, Ni, Zn, Mn, etc., are co-precipitated in
Fe oxides (Stumm and Morgan, 1981 ). Metals become associated
with iron oxides as a result of co-precipitation and adsorption
phenomena (Stumm and Morgan,1981 ). SEM-EDXA analysis shows
high C and O values with a higher incidence of Ca and other divalent
cations. This can lead to the formation of CaCO3 and other trace
metal carbonates. Carbonate formation can take place when bacterial production of bicarbonate alkalinity in sediments is substantial (ITRC, 2003). Carbonate precipitation is especially effective
for the removal of lead and nickel (Lin, 1995).
The elemental composition was determined by SEM-EDXA
analysis where two representative EDXA analyses were performed per sample and is provided in Table 2. The analysis showed
a common trend of high quantities of C in all the samples i.e. from
L1 to L3 indicating higher organic C (50e61%) in the sample that
also correlates with high COD, and BOD values analyzed during the
physico-chemical analysis. The C can also exist in the form of metal
carbonates as indicated in section 5. The N values ranged from 2.2
to 5.8% also indicating the presence of organic matter. Higher oxygen values ranging from 22 to 37% revealed organic matter and
minerals in the form of oxides and hydroxides. Among the cations,
Na predominated in all the leachate samples (5.8e12%). And among
the anions, Cl levels were relatively high (up to ~5%) compared to
other anionic radicals. The elemental composition of the leachate
solids is given in Table 2.

3.3. Statistical relationship
The data collected from various locations were analyzed with
Paleontological Statistics software (PAST 2.14). Correlation analysis
was performed and is elucidated in Table 3, and the level of significance is assessed at three different confidence intervals as
mentioned in Table 3. Firstly at high confidence levels (~99.9% i.e.
p < 0.001) Sulphide, Chloride and Fe are significantly correlated
with COD. Fe and sulphates correlated with alkalinity. The heavy
metals like Pb were significantly correlated with Cd. Ions as Na and
Cl were significantly correlated with Conductivity and the presence
of Ammo.-N respectively. Correlation at 99% and 95% can be viewed
from Table 3.

Multiparametric tests like detrended correspondence analysis
(DCA) help in reduction of the dimensionalities which is because of
a complex relationship of the species to the environment and the
physico-chemical parameters and helps in establishing linkages
through correlations between environmental, biological, and
chemical variables with the help of ordination axis. The steps to run
DCA have been provided in Appendix 1 in the supplementary
material. The detrended correspondence analysis indicated a
unimodal response of variance. Usually, such statistical analysis is
used to show affinities and differences between species and sites to
avoid the arc-shaped distribution of the samples when there is a
single strong gradient affecting the samples (Gauch, 1982). Some
environmental parameters in the form of physico-chemical variables were considered to interpret the patterns observed with DCA:
mainly biological and chemical variables in leachate were considered: nitrates, nitrites, ammonium, phosphates, BOD, COD total
bacterial and algal counts. The contribution of the environmental,
biological and chemical variables to explain species-sites variance
obtained in the DCA was analyzed by Pearson correlations using
environmental variables and values of the locations in the ordination Axes 1 and 2 in the multivariate analysis. Neither the environmental nor the biota data were transformed for the analysis.
Results from the DCA analysis for coverage values of samples
collected from the various locations are elucidated in Fig. 2. The
leachate samples are distributed along the plane defined by the two
first axes. Axes 1 and 2 account for 80.98% of the total variance of
the data set (73.61% and 7.37%, for axes 1 and 2, respectively). On
the first axis, samples were dominated by algal species i.e. Spirulina
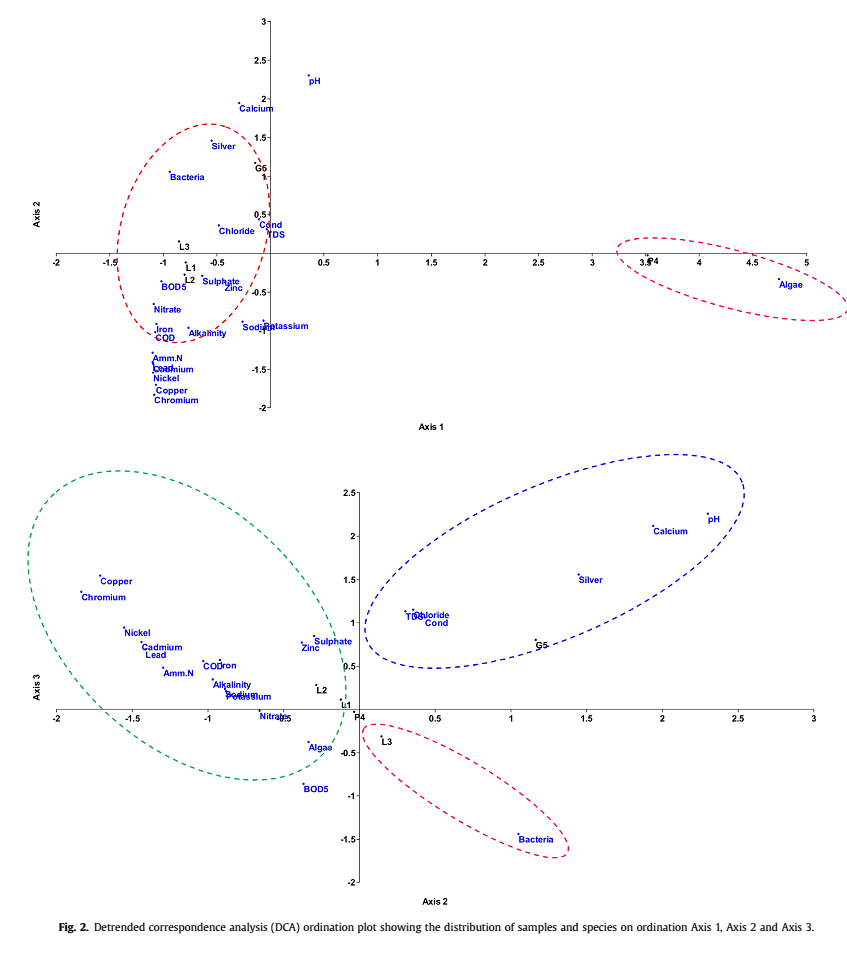
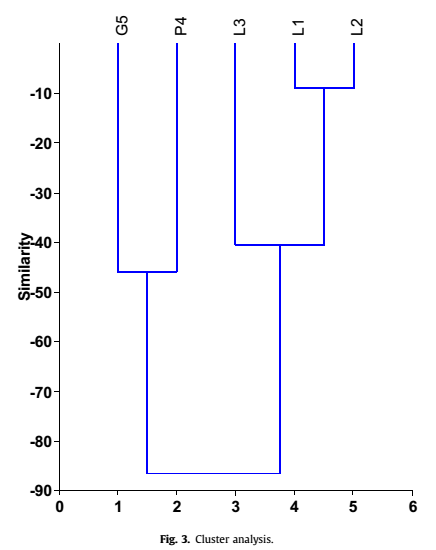
sp. collected from location 4 i.e. P4, situated close to the ordination
axis (Fig. 2), are opposed to samples dominated by bacteria. The
Axis 2 explains the variability of the bacterial population in relation
to L3. This explains higher bacterial abundance at L3. A big central
group is represented by the mostly the abundance of metals, with
other ionic parameters links to samples L1 and L2 are situated with
values between axis 2 to 3 (see Fig. 3).
The loadings on Axis 1 indicated that the axis is positively
impacted with location P4 and algal abundance. Similarly, loadings
on Axis 2 indicate strong correlations between location G5 with pH
and Ca values. However, loadings on Axis 3 indicate a high correlation between metals and other physico-chemical parameters and
negative correlations with the microbes. The cluster analysis helped in grouping the samples based on spatial similarities of the five
locations with varied concentrations and nature in the leachate
ponds, surface and ground water samples. The Wards method
showed two separate clusters that illustrate variations in the nature
and type of the samples based on (a) physico-chemical parameters
(b) trace metal concentrations and (c) biological sample abundance
and distribution. The results showed Cluster I (~55% similarity) comprised of two samples P-4 and G-5. Cluster II comprised of a
sub-cluster that consisting of samples L1 and L2 and a lone sample
L3. The sub-cluster and the lone sample L-3 were having ~60%
similarity. However, the samples within the sub-cluster that
comprise of L1 and L2 had a similarity value of> 90%. This indicates
L1 and L2 are more or less leachate sample of a similar nature while
leachate sample L3 is slightly different as it located a little away
from the landfi ll. This matches with the similarity of the samples
considering its physico-chemical characteristics. The L3 samples
have more organic matter and thus are different from the samples
L1 and L2. Contrary to these samples the G5 and P4 samples are
completely different. Also, G5 and P4 are different within the
cluster I. The cluster analysis shows a clear cut distinction between
the samples collected from the leachate ponds (L1-L3) and surface
and ground water (P4 and G5), that proves dissimilarity in their
nature. Such analysis helps in identifi cation of impacted sites for
better management practices.
3.4. Biological sample analysis
Biological analysis data are a more reliable assessment of longterm ecological changes in the quality of pond systems compared to
its rapidly changing physico-chemical characteristics that are faster
to analyze (Mahapatra, 2015). Biological indicators can portray the
changes in water bodies that help in understanding the systems
dynamics and aids in identifying key drivers by causal effect relationships (Mahapatra and Ramachandra, 2013; Mahapatra et al.,
2013b,c). Biological communities exposed to pollutants integrate
both past and present environmental phenomena.
The leachate samples collected from locations L1 to L3 were
studied through the scanning electron microscope. The results
showed (Fig. 4) that the leachate samples were dominated by
bacteria especially different kinds of bacillus i.e. individual bacillus
cells, diplo-bacillus and strepto-bacillus followed by coccus, spirochete and vibrio and the total bacterial count ranged from 3 to 4 log
orders. Some filamentous cyanobacteria were also observed in the
leachate samples. A complete bacterial analysis requires high
throughput bio-molecular tools or culture based assays. Classifi-
cation using advanced molecular analysis of the bacteria present in
leachate samples has been carried out by Zhang et al. (2011).
Detailed phylogenetic analysis of the bacterial population is presently being undertaken that will be communicated shortly.
However the samples collected from the Pond (P4), were prolifically dominated by Spirulina sp. with a very high cell count of
105 cells/ml. The abundance ofSpirulina was significantly correlated
with high ionic conductivity, pH and dissolved oxygen (Mahapatra
et al., 2013b). The sample collected from the openwell (G5) showed
(Fig. 4) low bacterial counts but revealed the presence of different
algal species mostly comprising of green algae and euglenoids. In
short the microbial analysis revealed myriads of bacterial populations mainly bacillus, coccus, and spirochete. Contrary to this the
surface water in the pond samples showed higher incidence of
single species of cyanobacteria i.e. Cyanophyceae indicating an
altogether different environment compared to the leachate sites.
The open well samples, however, showed different algal populations with low bacterial counts.
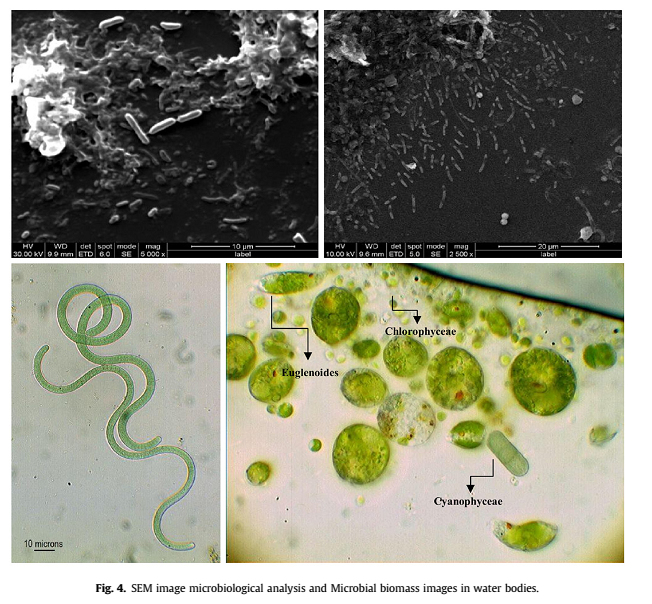
3.5. Leachate pollution index (LPI)
LPI values have been calculated for leachate samples of Mavallipura landfill site as per the procedure summarized in Table 4.
Mercury, arsenic, and cyanide have not been identified in the
leachate samples. Hence, no weightage for these trace metals were
provided for LPI calculation. In this study, a detailed analysis of total
coliform bacteria and phenolic compounds has not been carried
out. The highest leachate pollution index was observed in L1 owing
to potential toxicity and higher metal, inorganic and organics
concentrations. Significantly high ammo.-N and organic-N were
recorded in these samples pressing on immediate treatment for the
stalled leachate fractions in these MSW landfill sites. Earlier studies
have showed that high ammonia and alkalinity are toxic for duckweeds (Clement and Merlin, 1995). High N also poses a greater risk
of nutrient enrichment and consequent eutrophication in receiving
waters and is more harmful to aquatic animals in gaseous ammonia
form. The ammonia in the gaseous form produces odor problems in
the nearby area (Moreno et al., 2014). Generally, phenolic compounds are found to be very less in most of the Indian landfill
leachates (Devnita Polley, 2013). Thus in this study to bring out the
effect of background pollution index, LPI has been calculated.
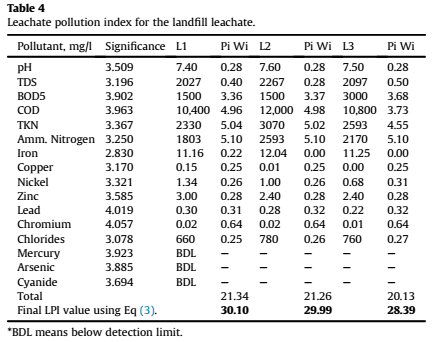
It can be seen that the LPI value for the L1 is the highest while
the LPI value for the L3 is found to be the lowest. Higher LPI presses
the need for treatment of Mavallipura landfill leachate, followed by
continuous monitoring. Aerobic biological treatment process with
extended aeration is required for treatment of Mavallipura leachate
as it has a high organic strength. The high ammo.-N can be treated
by nitrification followed by denitrification. Comparatively lower
values of LPI for L3 are attributable to low concentrations of heavy
metals in the leachate. However, the individual contaminants shall
meet the discharge standards before discharge of leachate into any
surrounding water bodies. The results indicate that the L1 and L2
have relatively high LPI value in comparison with the L3 and forms
a different group/cluster, evident from the cluster analysis and
therefore are not stabilized, with relatively high contamination
potential and needs physico-chemical and biological treatment to
prevent any further detrimental effects on surrounding eco-system
and water environment. Mavallipura leachate samples can, therefore, pose a threat to the environment and human health and hence, measures and continuous monitoring must be ensured.
Similar studies conducted on leachate samples (Devnita Polley,
2013) for Dhapa landfill site (KLS), Kolkata, India showed a relatively high LPI (40.32) on the other hand relative low LPI values of
~26 were observed (Slomczynska and Slomczynski, 2004) that
further decreases to ~7.03 upon treatment that was under
permissible limits.
3.6. Water quality index (WQI)
The surface water bodies near the Mavallipura landfill site are
important sources of water for human activities. Unprecedented
and continuous lobbying of MSW in the nearby landfill site can
affect the water quality and thus the health of the local community.
In the present purview of MSW disposal, with steep and unstable
slopes, there can be ample chances of leachate runoff to the low
lying water bodies. This also affects the ground water quality in the
immediate vicinity. Therefore, WQI that surrogates and weights the
water quality offers a useful representation of the overall quality of
water for public use, gauzing the appropriateness of the water for
further use and other utilitarian values. Table 5 shows the calculations for WQI values of pond (P4) and open well (G5) samples
near the same landfill area.
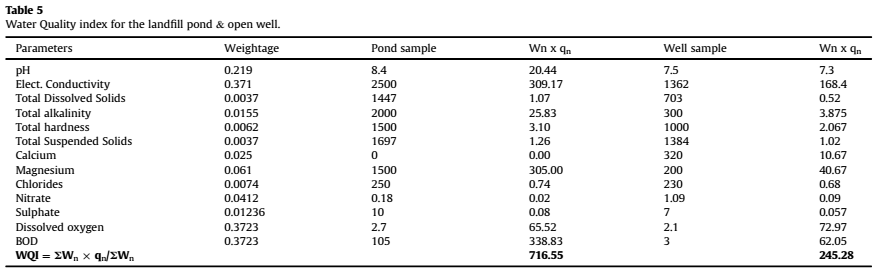
Water quality index of the present water body is established
from necessary physico-chemical parameters. The values of various
physico-chemical parameters for calculation of water quality index
are presented in Table 5. Based on earlier studies this water quality
rating clearly shows that the status of the water body is eutrophic,
and it is unsuitable for drinking and also observed that the pollution load is relatively high. Similar observations were recorded
earlier (Yogendra and Puttaiah, 2008), where low DO, high BOD,
and nitrates showed high WQI and thus nutrient enrichment in the
urban water body Gopishettykere, in Shimoga town, Karnataka.
High concentrations of sulphates, chlorides, and nitrates observed
from the present study indicate unsuitability of this water for domestic use.
The above water quality is also supported by the variations in
physiochemical parameters. Total dissolved solids and electrical
conductivity were found to be very high. Major anions like chloride
are one of the most important parameters in assessing the water
quality. The higher concentration of chlorides indicates a higher
degree of organic pollution. The concentration of dissolved oxygen
indicates the distribution of fl ora and fauna. Biochemical oxygen
demand (BOD) indicates the organic load in water bodies. Higher
BOD values are found in the polluted water. The results revealed
that quality of ground water resources in Mavallipura landfi ll is
deteriorating day by day; largely as a result of the poor practice of
solid waste management. Hence, an effective precautionary plan is
required for the sustainable management, which can be used as a
guideline in the regulation and supervision of ground water operations. The WQI values elucidate poor ground water in these areas
and necessitate immediate action and investigations for identifying
possible sources of contamination and consequential deterioration.
Moreover, proper management strategies and effective precautionary plans are required for the appropriate treatment and
management of solid waste that safeguards our future water
resources.
The present study highlights the present status and the quality
of the landfi ll leachate, through various characterization techniques
and shows high organic matter, inorganic nutrients and trace
metals in leachate that can potentially contaminate the surface and
the ground water resources precipitation through runoff and
leaching respectively. Diverse microbial population found in the
study can further screened for biological treatment of landfi ll
leachate. This study shows a need for better collection, containment and treatment of the landfi ll leachate to avoid environmental
externalities and health hazards that addresses sustainable waste
management in cities. Such type of studies would lead to devising
vital strategies with proper actions and management plan for
abating environmental pollution and safeguarding the future water
resources
|