 |
Bioremediation and lipid synthesis through mixotrophic algal consortia
in municipal wastewater |
 |
Durga Madhab Mahapatra a,b H.N. Chanakya b,c Ramachandra T V a,b,c,*
a Energy & Wetlands Research Group, Center for Ecological Sciences [CES],
b Centre for Sustainable Technologies (astra),
c Centre for infrastructure, Sustainable Transportation and Urban Planning [CiSTUP],
Indian Institute of Science, Bangalore, Karnataka, 560 012, India
*Corresponding author: cestvr@ces.iisc.ernet.in
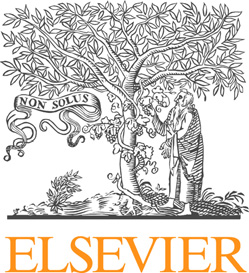 |
Citation : Durga Madhab Mahapatra, H N Chanakya, Ramachandra. T.V, Bioresource Technology, Volume xxx 2014, 1-9 pages.
Keywords : Mixotrophic algal consortia, Lipid, Wastewater treatment, GC–MS, SEM-EDXA
Publisher : Elsevier Ltd.
PDF |
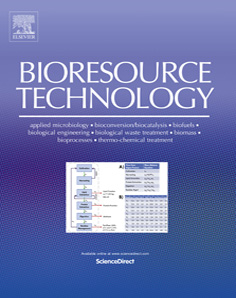 |
|
Results and Discussion
3.1. Assessment of nutrient uptake capability of algal consortia
The growth of the algal consortia monitored for 12 days as shown in Fig. 1a depicts the growth profile of mixed algae in wastewater. The algal consortia grew exponentially at a mean specific growth rate of 0.2/d in mixotrophic conditions. The consortia exhibited exponential growth (p < 0.05) with two distinct phases immediately after inoculation without lag phase. This is further substantiated by the similar decline pattern in TOC and TN due to algal growth with the uptake of nutrients. In the first phase, biomass increased from 0.17 ± 0.046 to 0.66 ± 0.042 g/l (30%) during the initial 4 days due to heterotrophy with the assimilation of easily available organic C. The second exponential phase, from day 6 to day 11, shows a biomass increase from 0.71 ± 0.037 to 1.64 ± 0.043 g/l (57%), which can be attributed to mixotrophy involving available residual organic C and dissolved CO2 that is significantly higher than the initial phase. The algal consortium predominantly comprising of Euglena spp., Phacus spp., Chlorella sp., Chlorococcum sp. and Phormidium sp. has played a decisive role in the assimilation of carbon. pH at the beginning of the experiment was 6.3 that indicated a slightly acidic media largely due to the presence of semi decomposed carbon substrates in the forms of acetate, propionate, butyrate etc. as reported earlier ( Zhou et al., 2012b). pH gradually increased to 8.6 with algal growth and a simultaneous increase in photosynthesis and accumulation of OH- ions.
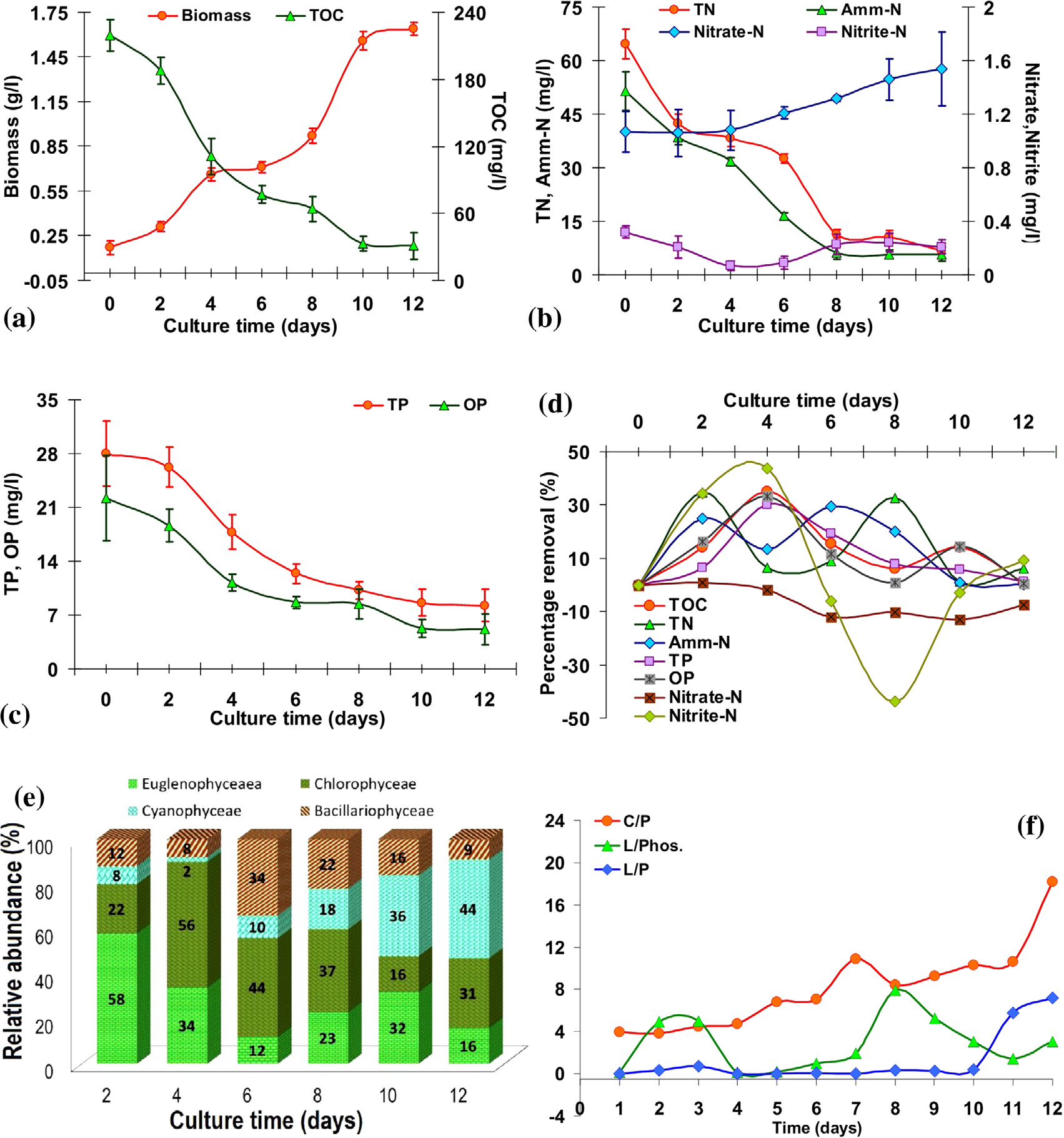
Fig. 1.
(a) Biomass growth and Total Organic Carbon (TOC) removal during culture; (b) TN, Amm.-N, nitrate-N and nitrite-N removal curve; (c) TP and OP removal by algal consortia during culture; (d) percentage nutrient removal of the mixed algal consortia during culture; (e) algal species composition variation provided as relative abundance during culture period; (f) ratio’s of macromolecular composition and their changes across the growth period. |
The biomass growth and productivities are shown in Table 1. Due to availability of abundant organic carbon, the biomass productivity was 122.5 mg/l/d in the reactor, which is higher than an earlier report (Chinnasamy et al., 2010) of 57 mg/l/d (in raceway pond) and 70 mg/l/d (mixed algal species cultured in poly bags). Compared to these, Chlorella minutissima grown in an optimised media showed a biomass productivity of 1780 mg/l/d ( Li et al., 2011) in suspended algal systems, which seems quite high and rare.
Table 1 - Specific growth rate and biomass productivity. |
Day |
Algal biomass dry wt. (g) |
μ |
σ |
Inc. growth (%) |
%age Growth |
SGR (/d) |
Biomass productivity (g/l/d) |
0 |
0.17 |
0.046 |
– |
– |
– |
– |
2 |
0.31 |
0.033 |
45.16 |
11.38 |
0.30 |
0.07 |
4 |
0.66 |
0.042 |
53.03 |
28.46 |
0.38 |
0.18 |
6 |
0.71 |
0.037 |
7.04 |
4.07 |
0.04 |
0.03 |
8 |
0.92 |
0.048 |
22.83 |
17.07 |
0.13 |
0.11 |
10 |
1.56 |
0.062 |
41.03 |
52.03 |
0.26 |
0.32 |
12 |
1.64 |
0.043 |
4.88 |
6.50 |
0.03 |
0.04 |
|
μ – mean; σ – standard deviation. |
TOC reduced from 219 ± 14 to 31 ± 12 mg/L in 12 days with a removal efficiency of ~86% due to an initial heterotrophic mechanism (with the abundant organic load). This was comparable to 86% carbon removal with Chlorella vulgaris (Feng et al., 2011), 98% carbon removal in mixed algal culture ( Prathima Devi et al., 2012) and ~36–57% carbon removal in diluted piggery wastewater (Wang and Lan, 2011). Incomplete removal of TOC could be attributed to growth inhibition due to either light limitation or presence of refractory carbon/algal polysaccharide secretions. Due to the filtration of cultured broth, visible suspended matter was found to be less (absorbance = 0.04) with ample light penetration during initial stages. Higher biomass densities (absorbance = 2.9) after the day 10 have contributed to a light restricted environment (with 5–7% light penetration) ceasing algal growth and consequent TOC removal. Specific growth rates differed from 0.38/d (initial 4 days) to 0.26/d (second phase corresponding to day 6 to day 12) that correlated with corresponding TOC removal of 219 to 111 mg/l (r = 0.9, p < 0.01) during the initial period and 77–33 mg/L (r = 0.99, p < 0.01) during the second phase of the exponential growth during algal culture.
Decline of TN from 64.6 ± 4.2 to 6.66 ± 2.1 mg/l (Table 2; Fig. 1b) during the growth period indicate ~90% of nitrogen assimilation (in the form of ammonium, ~80%) by growing algal cells in the presence of higher quantum of organic carbon. Nitrogen removal of 34.5% was observed during the first 2 days (heterotrophic phase) followed by 32.5% from day 6 to day 8 (mixotrophic phase) and overall removal of 82% was observed during all the 8 days (Fig. 1d). These were comparable to an earlier report (Cho et al., 2011) and they were also relatively higher compared to 78% TN removal of diluted primary wastewater and 54–75% of digested manure (Wang et al., 2010).
Table 2 : Decrease in nutrients and nutrient removal rates. |
Day |
TOC (mg/l) |
TN (mg/l) |
Amm.-N (mg/l) |
TP (mg/l) |
OP (mg/l) |
μ |
σ |
Inc. Rem. |
% Rem. |
μ |
σ |
Inc. Rem. |
% Rem. |
μ |
σ |
Inc. Rem. |
% Rem. |
μ |
σ |
Inc. Rem. |
% Rem. |
μ |
σ |
Inc. Rem. |
% Rem. |
0 |
219 |
14 |
– |
– |
64.6 |
4 |
– |
– |
51.5 |
5.5 |
– |
– |
28 |
4.3 |
– |
– |
22.2 |
2.4 |
– |
– |
2 |
188 |
12 |
14.16 |
12.16 |
42.3 |
2.6 |
34.52 |
76.90 |
38.6 |
2.12 |
25.05 |
49.62 |
26.2 |
2.26 |
6.43 |
8.18 |
18.6 |
1.64 |
16.22 |
22.50 |
4 |
111 |
16 |
40.96 |
30.20 |
38.2 |
2.22 |
9.69 |
14.14 |
31.7 |
1.11 |
17.88 |
26.54 |
17.8 |
1.64 |
32.06 |
38.18 |
11.24 |
1.77 |
39.57 |
46.00 |
6 |
77 |
8 |
30.63 |
13.33 |
32.5 |
1.26 |
14.92 |
19.66 |
16.5 |
0.76 |
47.95 |
58.46 |
12.4 |
1.77 |
30.34 |
24.55 |
8.66 |
0.26 |
22.95 |
16.13 |
8 |
64 |
11 |
16.88 |
5.10 |
11.5 |
1.11 |
64.62 |
72.41 |
6.24 |
1.89 |
62.18 |
39.46 |
10.22 |
2.43 |
17.58 |
9.91 |
8.44 |
0.69 |
2.54 |
1.38 |
10 |
33 |
7 |
48.44 |
12.16 |
10.66 |
1.76 |
7.30 |
2.90 |
5.77 |
1.17 |
7.53 |
1.81 |
8.62 |
1.08 |
15.66 |
7.27 |
5.26 |
1.04 |
37.68 |
19.88 |
12 |
31 |
12 |
6.06 |
0.78 |
6.66 |
2 |
37.52 |
13.79 |
5.76 |
2 |
0.17 |
0.04 |
8.26 |
2.4 |
4.18 |
1.64 |
5.13 |
1.67 |
2.47 |
0.81 |
|
μ – mean; σ – standard deviation. |
NH4-N had dropped from 51.5 ± 5.5 to 5.76 ± 2 mg/l (89% removal) during 12 days of batch experiment (Table 2; Fig. 1b). This was lower compared to the values in the earlier studies carried out with Chlorella vulgaris in artificial wastewater (97% NH4-N removal) conducted in heterotrophic/mixotrophic mode (Feng et al., 2011), and 96% removal in mixed algal culture grown in municipal wastewaters (Woertz et al., 2009). The incomplete removal of NH4-N could have been due to carbon limitation or light interception as dense algal cultures were floating (of large and filamentous algae Spirulina and Phormidium, respectively) thereby interrupting light penetration. However, the current report of NH4-N removal is better than the reported NH4-N removal (69%) by Auxenochlorella protothecoides UMN280 grown in municipal wastewaters (Zhou et al., 2012b).
The average C/N and N/P ratio of the media were 3.77 ± 1.14 and 1.69 ± 0.67 respectively that favoured nitrogen removal. The decrease in nitrogen values correlated with the increased biomass (r = −0.9; p < 0.005) indicating nitrogen assimilation during algal growth. Highest NH4-N removal was observed during the first 2 days (25.04%) and from day 6 to 8 (29.51%), which were similar to nitrogen removal ( Fig. 1d).
NH4-N removal happens due to ammonia volatilisation under high pH and temperature. However, in the present study the major fraction of NH4-N removal was due to assimilation by algae as pH and temperature were moderate (pH was <8.5 and temperature was 26 ± 4 °C) in the reactor. Fig. 1b shows a gradual increase of nitrate-N concentrations from1.07 ± 0.15 to 1.54 ± 0.28 mg/l due to nitrification. This was lower than 67% of nitrate-N removal in wastewater through mixed algal cultures isolated from wastewaters (Prathima Devi et al., 2012). On the other hand, Nitrite-N decreased slightly from 0.32 ± 0.05 to 0.21 ± 0.05 mg/l due to rapid assimilation by algae and slower nitrification process.
TP values listed in Table 2 showed a decrease from 28 ± 4.3 to 8.26 ± 2.4 mg/l (70% removal) compared to ~72% (Wang et al., 2010), 31–77% (Wang and Lan, 2011), ~84% removal (Cho et al., 2011); ~90% (Feng et al., 2011, Zhou et al., 2012a and Zhou et al., 2012b) and ~95% (Yujie et al., 2011). Phosphorus concentration in wastewater was relatively higher (Fig. 1c) than that of carbon and nitrogen (C:N:P = 7.8:2.3:1). Algae used only a small fraction of phosphorus that was available in the system, as a result of which ~30% of phosphorus remained residual in the culture. This fraction was unutilised as algal growth reached saturation due to carbon and nitrogen limitations as well as light limitations in the dense algal culture. Higher phosphorus removal on day 4 (30%) coincided with carbon assimilation that had resulted in a higher biomass growth (Fig. 1d). Excess phosphorus was also revealed by a lower N/P ratio (range 0.8–2.6) of 1.69 ± 0.67, which had not affected either biomass growth or productivity. Ortho phosphate removal (Fig. 1c) efficiency was 78% (22.2 ± 2.4 to 5.13 ± 1.67 mg/l) that was relatively higher than phosphates removal (40–65%) by mixed algal cultures grown in wastewater (Prathima Devi et al., 2012). Higher OP removal (33.15%) had occurred on day 4 (Fig. 1d). Organic phosphates varied from 7.6 to 1.78 mg/l, which constituted to about 16% of TP. Fig. 1c highlights this decline of TP that reached a minimum of ~2 mg/L (on day 8, after which, it stabilised to ~3 mg/l). The conversion of organic phosphorus into inorganic forms aided in the assimilation of phosphorous by algae.
The species composition and dynamics are elucidated in Fig. 1e. Euglenophyceae members such as Euglena sp., Phacus sp. etc. dominated (58%) during the early culture period up to the first 4 days that coincided with carbon uptake and higher biomass growth. In high organic environment, euglenoids grow in heterotrophic mode ( Mahapatra et al., 2013a, Mahapatra et al., 2013b and Ramachandra et al., 2013). Chlorophyceae (especially Chlorella sp. and Chlorococum sp.) increased from 22% to 56% (day 4), and then, showed a declining trend. Mixotrophic chlorophycean members (Chlorococcum sp.) are known to grow faster in nutrient rich conditions ( Mahapatra and Ramachandra, 2013).
Bacillariophyceae (Cyclotella sp. and Nitzschia sp.) considered as facultative heterotrophs ( Mahapatra et al., 2013b) increased to 34% (day 6) and showed a gradual decline to 9% (day 12). The species turnover indicated changes in nutrient availability and reactor conditions that was suitable for assimilation and growth of chlorophyceae and bacillariophyceae members resulting in switch over from heterotrophy to mixotrophy. Cyanophyceae (blue green algae as Phormidium sp. (filamentous) and Spirulina sp. (spiral shaped)) showed an increasing trend ( Fig. 1e) from 2% (day 4) to 44% (day 12). These algae are often found as naturally floating (due to dense gas vacuoles) masses in urban lakes and wastewater treatment lagoons ( Mahapatra et al., 2013b). The dense gas vacuoles in filamentous algae help in floatation. This highlighted that algal consortia of varied proportion depending on the nutrient availability enables the treatment of wastewater. Diverse species with differential interactions/competition also contributes to the system stability with enhanced biomass growth and efficient removal of nutrients. This required further investigation, which is being undertaken.
3.2. Mechanisms for optimal harvesting of algae – algal floc formation, P accumulation and elemental composition
SEM-EDXA analysis of suspended algal biomass revealed higher organic content comprising ~55% C; 7% N with a higher P accumulation of ~4% (Fig. S1 provided in the supplementary material). Higher phosphorus accumulation (in the form of Poly-P; Chen et al., 2011) in this biomass was due to algal habitat that stored enormous phosphorus which was coupled with a higher metabolic phosphorus demand to fulfill the cellular phosphorus requirement. Further, phosphorus fractionation was required to understand the metabolic phosphorus demand and phosphorus storage, which would help in estimating phosphorus holding capacity and its relevance to phosphorus recovery and recycling in the environment. In the present case, phosphorus accumulation in the biomass was attributed to higher ortho phosphate concentration remaining in the media (~22%) that had not precipitated out because of low alkaline conditions. In the present study, the suspended matter were mostly in the form of granules (15–45 μm) that comprised of ~50% C and ~33% oxygen with higher levels of Ca (~5%) indicating the formation of CaCO3 salts that get adsorbed over other organic granules or over biogenic Si (from diatoms) in the reactor. The reactor sludge in the final stages showed abundant organic matter with inorganic elements like Si, Al, Mg etc.
The analysis of elemental composition of the algal flocs/scum (helpful in algal harvest), floating on the reactor surface and the surface morphology (Fig. S1 provided in the supplementary material), was done through SEM-EDXA. Smaller flocs (<0.5 cm) mainly composed of green algal cells were flocculated by probable algal derived flocculant (unpublished data) in combination with metal ion. Algal flocculation was due to the negative charges on the cell surfaces during the peak period of algal growth (Chen et al., 2011). Floc formations or auto-flocculation (Salim et al., 2011) have been attributed to pH transitions (Wu et al., 2012) and availability of higher cations (divalent) which lead to mineralisation at the mature stages of algal growth. Filamentous cyanophycean masses as Phormidium sp. (1–4 cm diameter) with gas bubbles float in large masses on the surface. This natural phenomenon of aggregation and floatation was useful for efficient algal harvesting. Harvesting of algae has been a major constraint for its commercial use as existing mechano-chemical processes are energy and also time consuming when compared to bio flocculation (Park et al., 2012). Therefore, there were attempts to harvest algal biomass through bio-flocculation involving algal-bacterial interaction ( Su et al., 2011) and fungal palletisation-assisted bio-flocculation of algal cells ( Zhou et al., 2012a). In such cases, the culture environment plays a major role in phase separations in flocculation and sedimentation.
Table 3 lists the elemental composition of Algal scum (flocs) and other substrates (reactor sludge, naive algal biomass and suspended matter). The elemental analysis showed high concentration of Ca (~26%), Fe (~5%), K (~8%) and Cl (~8%) in the algal surface flocs. However, these elements were low in other substrates as they were primarily organic. The elemental peaks indicated the presence of Ca and P (Fig. S1 provided in supplementary material). Higher froth or algal floc formations had been attributed to Ca, Al, Cl (Chen et al., 2011, Park et al., 2012 and Wu et al., 2012). Phosphorus helps in coagulation while CaCO3 (calcium, carbon and oxygen) and FeCl3 (due to higher chlorine and iron) aid in the formation of flocks in algal scum.
Table 3 : SEM EDXA elemental analysis of algal biomass, flocs, suspended matter and reactor sludge. |
|
C |
N |
O |
P |
S |
Cl |
K |
Ca |
Fe |
Na |
Mg |
Al |
Si |
F |
Total |
Algal scum |
36.97 |
0.01 |
10.64 |
2.36 |
2.72 |
7.85 |
8.31 |
26.31 |
4.83 |
– |
– |
– |
– |
– |
100 |
Reactor sludge |
61.39 |
3.45 |
17.65 |
2.32 |
0.61 |
3.7 |
0.63 |
5.54 |
– |
0.99 |
1.81 |
0.56 |
1.35 |
– |
100 |
Algal biomass |
69.49 |
6.7 |
14.77 |
3.54 |
1.69 |
– |
0.58 |
2.69 |
– |
– |
0.54 |
– |
– |
– |
100 |
Suspended matter |
50.64 |
– |
32.72 |
2.53 |
0.63 |
– |
0.07 |
5.25 |
1.61 |
0.25 |
0.15 |
1.97 |
2.54 |
1.64 |
100 |
|
SEM studies highlighted diverse surface morphologies of algae with vast surface area that helped in adsorption, and uptake of elements. Algal cell surface with extracellular polymeric secretions (mucilage) bind the cells together, while the gas vacuoles aid in floatation and auto flocculation. Surface complexation by attachment of metals on cell surfaces also helped in floc formation. However, this required further research focussing on surface morphologies, texture and ionic interactions with cells surfaces.
3.3. FTIR analysis
ATR-FTIR spectra of the algal consortia showed five distinct absorption bands (corresponding to wave numbers 1800–800 cm−1) representing specific functional groups as per biochemical standards (Stehfest et al., 2005). FTIR spectrogram of the algal consortia showed the quantitative changes in the macromolecular compositions with respect to the nutrient content, etc. during the culture period. The algal consortia showed significant variation in the carbohydrate, protein, lipid and phosphate contents (Table T1, Supplementary material) with an increase in L/P ratio during the final stages.
The daily observations of the variations in cellular macromolecular composition (Table T1) and Fig. 1f highlighted a peak for carbohydrate/amide (C/P) ratio on day 7 and day 12 (highest), inferring a gradual increase in polysaccharides with TOC assimilation. High C/P ratio indicated a net decrease in the protein content (with TN and NH4-N removal, as discussed earlier) and the synthesis of polymeric carbon secretions helped in aggregation of algae during floc formation. This further demonstrated the potential NH4-N limitations in triggering the lipid synthesis and accumulation. In the present experiment, the C/P ratio of the algal consortia showed 4.58-fold increase that varied from 3.95 to 18.15 on day 12. This was higher compared to an increase of 1.14 and 2.24 times in Phaedactylum tricornitum ( Stehfest et al., 2005) and lower than 7- and 9.6-fold increase in Chlamydomonas reinhardtii ( Dean et al., 2010) and Microcystis auregonosa ( Stehfest et al., 2005) respectively. Nitrogen starvation leads to diversion of carboxyl part (precursor to protein) to lipid and polysaccharides enhancing C/P values. This is in contrast to low C/P (0.6) in Pediastrum duplex under natural conditions ( Dean et al., 2012).
Lipid to amide (L/P) ratio increased on day 3 and reached threshold on day 10 as the culture inched towards the stationary phase (Fig. 1f) due to accumulation of lipid at the expense of protein. L/P ratio increased from 0.014 to 3.08 during the culture period and was higher compared to 1.27- and 2.42-fold under nutrient sufficient and deficient conditions in Phaedactylum tricornitum ( Stehfest et al., 2005), 1.5-fold in Scenedesmus subspicatus ( Dean et al., 2010) and 6.75-fold in Chlamydomonas reinhardtii ( Dean et al., 2010). However, Pediastrum duplex under natural conditions, showed a minimal L/P of 0.2 ( Dean et al., 2012). These results further substantiated that lipid accumulation was a function of nutrient deprivation at an advanced stage of the cell culture.
Increased lipid/phosphates (L/Phos.) exhibit bimodal pattern with the first peak during the initial 4 days and the second one spanning from day 7 to 10 (Fig. 1f). Phaeodactylum tricornitum had L/Phos ratio increased by 1.23 and 1.2 times under nutrient sufficient and stress conditions on day 14 ( Stehfest et al., 2005).
3.4. total lipid content, productivity, potential yield and FAME composition
Algae grown in carbon rich wastewater stored more lipids through heterotrophy (Bhatnagar et al., 2010). Organic and inorganic forms of carbon get transformed into algal biomass primarily due to mixotrophy and subsequently get accumulated as lipids. Total lipid in the current study was significantly contributed by the dominant algae -euglenoids, chlorococcales and Phormidium sp. as had been reported earlier ( Ramachandra et al., 2013 and Mahapatra and Ramachandra, 2013). Maximum total lipid content of the algal consortia was 28.5%, which was higher compared to 16% in Chlorella minutissima UTEX2341 grown in optimised media ( Li et al., 2011), 20–42% in Chlorella vulgaris grown in artificial wastewaters ( Feng et al., 2011) and 12–80% in mixed algae grown in untreated carpet mill effluents ( Chinnasamy et al., 2010).
Algal consortia of euglenoids, chlorococcales and Phormidium sp. were suitable to treat Indian municipal wastewaters at a decentralised level. Higher biomass growth with moderate lipid content (28.5%) amounted to lipid productivity of ~31.77 mg/l/d suggesting higher lipid content with biomass increase. This was higher compared to 24 mg/l/d in dairy and municipal wastewaters ( Woertz et al., 2009), ~23 mg/l/d in wastewater effluent ( Cho et al., 2011), 4–4.5 mg/l/d in wastewater ( Chinnasamy et al., 2010); 2–6 mg/l/d in Chlorella pyrenoidosa ( Wang and Lan, 2011); 7–23 mg/l/d in filtered secondary treated wastewater ( Cho et al., 2011) and lower compared to 40 mg/l/d from Chlorella vulgaris grown in artificial wastewater ( Yujie et al., 2011), 44–147 mg/l/d in a semi-continuous system ( Feng et al., 2011) and 200 mg/l/d in Chlamydomonas reinhardtii grown in concentrated wastewater ( Li et al., 2011).
The surface productivity of algal biomass was 6.36 g/m2/d accounting to a lipid productivity of ~19.08 tonnes/hectare (Ha)/yr (or 17.69 kl/Ha/yr considering 300 sunny days per year (as in Bangalore), which was higher compared to 11 kl/Ha/yr (Woertz et al., 2009). However, this yield depends on outdoor conditions like the season, temperature, nutrient availability as well as predation by zooplanktons and grazers, seasonal algal succession, wash-outs, etc. The utility of algal fuel depends on lipid and thermal characteristics.
Table 4 lists the percentage composition of respective FAME with the time of retention, peak area, and percentage correlation maxima. Composition of methyl esters in lipids in addition to the chemical characteristics like, number of carbon atoms, degree of saturation/unsaturation etc. governs the quality of biofuel (Ramachandra et al., 2009). Eighteen different fatty acids were identified in the FAME mixture extracted from wastewater algal consortia. The lipid profile of the algae showed a dominance of C16:0 and C18:0 methyl esters, and moreover, greater than 91% of the FAME comprised of C16 to C18 FAME indicating good quality biofuel (Table 4). C18 fatty acids are very important from a fuel perspective (Cho et al., 2011) as it is an integral part of vegetative oil comprising of stearic, oleic, linoleic and linolenic acids. However, <25% of unsaturated fatty acids was recorded in the FAME mix. Higher abundance of saturates as palmitate and stearate signify better fuel properties with smooth ignition (Feng et al., 2011) and higher cetane values which provide good combustion with lower emissions (Li et al., 2011). The unsaturated fraction of biodiesel is useful for achieving increased cold filter plugging point and hence higher stability at low temperatures. The susceptibility of polymerisation (Venkata Mohan et al., 2011) and oxidation of fuel (Damiani et al., 2010) during storage is low as the algal oil has low PUFA (∼10%) content. The FAME composition of algal consortia (in the present study) follows an order, with major contributions from saturates as palmitic acid C16:0 (42.30%) > stearic acid C18:0 (25.69%) > unsaturates as oleic acid C18:1(11) (10.87%) > C18:2(9) (5.27%). This is comparable to algae grown heterotrophically in piggery wastewaters with – Chlorella pyrenoidosa C18:2(30%) > C16:0(28%) > C18:3(27%) > C16:1(10%) ( Wang and Lan, 2011) and Chlorella minutissima UTEX2341 C18:1(9) (46.13%) > C18:2 (26.7%) > C16:0 (13.5%) > C18:0 (3.4%) and 16% lipid ( Li et al., 2011). About 76% of the total FAME comprised of saturated fatty acids contrary to studies ( Li et al., 2011 and Wang and Lan, 2011) that showed the dominance of unsaturated fatty acids. This was due to the extent of light exposure or high light intensities with larger dark periods that influenced nature and quality of fatty acids ( Su et al., 2011 and Seyfabadi et al., 2011). Culture experiments were conducted in outdoor open reactors with a 12 h light: 12 h dark photoperiods and the lipid profile showed that fatty acids with ~84% contributions from saturated (C16:0, C18:0) and unsaturated (C18:1, C18:2) fatty acids that are considered key components for biodiesel.
Table 4 : Percentage composition of FAME with the retention time in columns. |
No. |
FAME (chemical name) |
Chemical formula |
Retention time |
Peak height (×106) |
Corr. area (×106) |
Corr. % Max. |
% FAME |
1 |
Butanedioic acid, dimethyl ester |
C6:0 |
7.49 |
2.54 |
10.86 |
6.51 |
2.76 |
2 |
Methyl tetradecanoate |
C14:0 |
24.92 |
0.75 |
3.31 |
1.99 |
0.84 |
3 |
Tetradecanoic acid, 12-methyl ester |
C14:0-12 CH3 |
26.91 |
1.12 |
6.33 |
3.79 |
1.61 |
4 |
Methyl 4,7,10,13-Hexadecatetraenoate |
C16:4(4,7,10,13) |
29.99 |
1.19 |
7.49 |
4.49 |
1.90 |
5 |
7,10-Hexadecadienoic acid, methyl ester |
C16:2(7,10) |
30.27 |
0.99 |
4.50 |
2.7 |
1.14 |
6 |
7,10,13-Hexadectrienoic acid, methyl ester |
C16:3(7,10,13) |
30.47 |
1.33 |
6.57 |
3.94 |
1.67 |
7 |
7-Hexadecenoic acid, methyl ester |
C16:1(7) |
30.66 |
1.32 |
5.43 |
3.26 |
1.38 |
8 |
Hexadecanoic acid, methyl ester |
C16:0 |
31.61 |
12.88 |
167 |
100 |
42.30 |
9 |
Heptadecanoic acid, methyl ester |
C17:0 |
34.42 |
0.49 |
3.72 |
2.23 |
0.95 |
10 |
8,11,14-Eicosatrienoic acid, methyl ester |
C18:3(8,11,14) |
35.92 |
0.06 |
0.19 |
0.12 |
0.05 |
11 |
Methyl Octadecatetraenoate |
C18:4(6,9,12,15) |
35.94 |
0.28 |
1.19 |
0.71 |
0.30 |
12 |
9,12-Octadecadienoic acid, methyl ester |
C18:2(9,12) |
36.49 |
3.72 |
20.78 |
12.46 |
5.27 |
13 |
9-Octadecadienoic acid (Z)-metyl ester |
C18:1(9) |
36.74 |
7.30 |
42.85 |
25.7 |
10.87 |
14 |
11-Octadecadienoic acid, metyl ester |
C18:1(11) |
36.84 |
1.85 |
5.59 |
3.36 |
1.42 |
11 |
Octadecanoic acid, methyl ester |
C18:0 |
37.63 |
10.9 |
101 |
60.73 |
25.69 |
14 |
Eicosanoic acid, methyl ester |
C20:0 |
43.08 |
0.35 |
1.66 |
1 |
0.42 |
15 |
Docosanoic acid, methyl ester |
C22:0 |
48.39 |
0.35 |
1.48 |
0.89 |
0.38 |
16 |
Tetracosanoic acid, methyl ester |
C24:0 |
51.69 |
0.51 |
1.42 |
0.85 |
0.36 |
17 |
Hexadecanoic acid, methyl ester |
C26:0 |
53.80 |
0.48 |
1.51 |
0.91 |
0.38 |
18 |
Octacosanoic acid, methyl ester |
C28:0 |
55.52 |
0.46 |
1.28 |
0.77 |
0.32 |
|
|
Saturates |
|
|
|
|
|
76.00 |
|
Unsaturates |
|
|
|
|
|
24.00 |
|
Monounsaturated FA |
|
|
|
|
|
13.67 |
|
Polyunsaturated FA |
|
|
|
|
|
10.33 |
|
Unsaturate to Saturate ratio |
|
|
|
|
|
0.32 |
|
C16–C18 |
|
|
|
|
|
91.04 |
|
3.5. DSC analysis
Thermal analysis through DSC provides quantitative information about the substrate from the perspective of energy generation. Thermal decomposition was assessed through thermogram for (a) algal matter, (b) spent algal matter after lipid extraction, (c) algal mass floc floating on the surface of the reactor and (d) reactor bottom sludge. This comprised of three different stages (i) initial stage (5 °C to ~175 °C) characterised by a large endothermic peak near 80 °C, corresponding to dehydration (Salmon et al., 2009), (ii) intermediate stage (175 °C to 350 °C) comprising of broad exothermic peak due to thermal decomposition of polymers as carbohydrates/lipids and to certain extent decarboxylation of carboxylic groups and aliphatic group dehydration (Silva et al., 2012) and (iii) final stage (at 350 °C to >500 °C) comprising of thermal degradation of aromatic substances (Sostaric et al., 2012 and Silva et al., 2012), with a large hike in the exothermic region (and peak >500 °C). Algae sample with two exothermic peaks jointly accounted for 123.4 J/g, which was higher than that of Chlorella vulgaris (82 J/g), ( Sostaric et al., 2012) and sewage sludge (11.2 J/g) ( Silva et al., 2012).
Algal biomass and spent biomass after extraction showed better thermal properties as compared to the surface algal floc and algal sludge (less peaks between 175 and 350 °C). However, the reactor sludge sample and algal spent biomass (after extraction) showed increased heat flow beyond 380 °C due to net decrease in the carbohydrate content with increased molar weight, stability and more aromatic conversions of the organic content. Algae and spent algal biomass (extract) after cell disruption and lipid extraction showed a significant difference in the exothermic peaks (p < 0.01) among various substrates considered for the study, suggesting scope for energy derivation with various by-products of the reactor and algal spent biomass.
|
|
|
|